Single Photon Generation and Spectroscopy Using Quantum Dots on Optical Nanofibers
全文
(2) Single Photon Generation and Spectroscopy Using Quantum Dots on Optical Nanofibers. by Ramachandrarao Yalla. A dissertation submitted in partial fulfillment of the requirements for the degree of. Doctor of Philosophy in the subject of. Physics to. Department of Applied Physics and Chemistry Graduate School of Electro-Communications The University of Electro-Communications Chofu-Shi, Tokyo, Japan December 2012.
(3) Single Photon Generation and Spectroscopy Using Quantum Dots on Optical Nanofibers. APPROVED BY SUPERVISORY COMMITTEE: CHAIRPERSON: PROF. Kohzo Hakuta MEMBER: PROF. Kouichi Yamaguchi MEMBER: PROF. Kenichi Nakagawa MEMBER: ASSOCIATE PROF. Yasushi Ofuchi MEMBER: ASSOCIATE PROF. Hideo Isshiki.
(4) c Copyright by Ramachandrarao Yalla 2012 All Rights Reserved.
(5) Dedicated to my Parents (Amma, Nanna, Pedananna).
(6) Abstract This thesis presents single photon generation and spectroscopy using quantum dots (q-dots) on optical nanofibers. Optical nanofibers are essentially vacuum-clad silica fibers with sub-wavelength diameter, which can support only the propagation of the fundamental mode through the guided mode. Optical nanofibers are realized using adiabatically tapered single mode optical fibers. Experiments were performed by depositing colloidal core-shell type CdSeTe (ZnS) q-dots on the optical nanofiber surface. A method is developed to deposit single q-dots systematically and reproducibly along the optical nanofiber. In the view point of investigation of fluorescence photon emission characteristics for single q-dots on optical nanofibers, the absolute fluorescence photon-count rates channeled into the nanofiber guided modes and the normalized photon correlations at various excitation laser intensities are measured. The results are able to obtain the product between the fluorescence photon channeling efficiency and the quantum efficiency of the single q-dots. However, the channeling efficiency cannot be determined without accurate information on the quantum efficiency of the single q-dots. Therefore, a method is devised to determine the channeling of fluorescence photons from single q-dots into the guided modes by measuring the fluorescence photon-count rates through the guided and radiation modes simultaneously. By measuring for various nanofiber diameters, a maximum channeling efficiency obtained to be 22.0 (±4.8)% at a fiber diameter of 350 nm for an emission wavelength of 780 nm. The measured results completely reproduce the theoretical predictions within the experimental error. We demonstrate in situ measurements of fluorescence quantum efficiency of single q-dots using the present nanofiber technique. We determine fluorescence quantum efficiency of single q-dots accurately by assuming the theoretical values for the channeling efficiency from the product of the channeling efficiency and the quantum efficiency. We demonstrate that one can pick up one q-dot on the nanofiber with fluorescence quantum efficiency higher than the average value measured for an ensemble..
(7) Thus, the optical nanofiber method offers a promising way for generating and manipulating single photons. This work should provide a basis for extending the optical nanofiber method to a general technique in quantum optics, which may open new possibilities in quantum information technologies..
(8) Table of Contents. List of Tables. vi. List of Figures. vii. 1 Introduction. 1. 1.1. Introduction . . . . . . . . . . . . . . . . . . . . . . . . . . . . . . . .. 1. 1.2. Optical nanofibers for quantum optics . . . . . . . . . . . . . . . . . .. 2. 1.2.1. Confinement of the field around the optical nanofiber . . . . .. 3. 1.2.2. Modified spontaneous emission of a quantum emitter around the optical nanofiber . . . . . . . . . . . . . . . . . . . . . . .. 4. Optical nanofibers as a tool for fluorescence measurements . .. 6. Summary of thesis . . . . . . . . . . . . . . . . . . . . . . . . . . . .. 7. 1.2.3 1.3. 2 Experimental Techniques 2.1. Experimental realization of optical nanofibers . . . . . . . . . . . . . 2.1.1. 2.2. 9 9. Characteristics of optical nanofibers . . . . . . . . . . . . . . .. 12. Precise spatial control of q-dots on optical nanofibers . . . . . . . . .. 14. 2.2.1. Semiconductor q-dots . . . . . . . . . . . . . . . . . . . . . . .. 14. 2.2.2. Subpicoliter needle dispenser . . . . . . . . . . . . . . . . . . .. 15. i.
(9) 2.3. 2.2.3. Inverted microscope system . . . . . . . . . . . . . . . . . . .. 16. 2.2.4. Procedure for depositing q-dots on the nanofiber surface . . .. 17. Procedure for fluorescence photon measurements . . . . . . . . . . . .. 19. 2.3.1. Avalanche photo-diodes. . . . . . . . . . . . . . . . . . . . . .. 20. 2.3.2. Counting board. . . . . . . . . . . . . . . . . . . . . . . . . .. 21. 2.3.3. Time-correlated single photon-counting system . . . . . . . . .. 21. 2.3.4. Optical-multichannel analyzer . . . . . . . . . . . . . . . . . .. 21. 3 Fluorescence Measurements through the Guided modes of Optical Nanofiber. 22. 3.1. Introduction . . . . . . . . . . . . . . . . . . . . . . . . . . . . . . . .. 22. 3.2. Experimental procedures . . . . . . . . . . . . . . . . . . . . . . . . .. 23. 3.2.1. Quantum dots deposition on the optical nanofiber . . . . . . .. 23. 3.2.2. Fluorescence photon measurements procedures through the guided modes . . . . . . . . . . . . . . . . . . . . . . . . . . . . . . .. 24. Measurement of light-transmission factor for guided modes . .. 25. Emission behavior of q-dots on optical nanofibers . . . . . . . . . . .. 26. 3.3.1. Spatial distribution of deposited q-dots . . . . . . . . . . . . .. 26. 3.3.2. Single/Two q-dots fluorescence photons statistics . . . . . . .. 27. 3.3.3. Emission spectrum of a single q-dot . . . . . . . . . . . . . . .. 30. Single/Two q-dots photon statistics data analysis . . . . . . . . . . .. 31. 3.4.1. Theoretical model for photon emission process of q-dots . . . .. 31. 3.4.2. Intensity dependence of photon correlation recovery rates . . .. 33. 3.4.3. Photon-count rate histogram . . . . . . . . . . . . . . . . . . .. 34. 3.4.4. Intensity dependence of photon-count rates . . . . . . . . . . .. 35. 3.5. Parameters for q-dots on optical nanofibers . . . . . . . . . . . . . . .. 37. 3.6. Discussion . . . . . . . . . . . . . . . . . . . . . . . . . . . . . . . . .. 37. 3.7. Conclusion . . . . . . . . . . . . . . . . . . . . . . . . . . . . . . . . .. 38. 3.2.3 3.3. 3.4. 4 Efficient Channeling of Fluorescence Photons into the Guided modes of Optical Nanofiber. 39.
(10) 4.1. Introduction . . . . . . . . . . . . . . . . . . . . . . . . . . . . . . . .. 39. 4.2. Experimental procedures . . . . . . . . . . . . . . . . . . . . . . . . .. 40. 4.2.1. Single/Two q-dots deposition at various nanofiber diameters .. 41. 4.2.2. Single/Two q-dots fluorescence photon measurements procedures 41. 4.2.3. Measurement of fluorescence light-transmission factors for guided and radiation modes . . . . . . . . . . . . . . . . . . . . . . .. 43. 4.3. Channeling efficiency into the nanofiber guided modes . . . . . . . . .. 44. 4.4. Effect of nanofiber as a cylindrical lens . . . . . . . . . . . . . . . . .. 45. 4.5. Deposition of single/two q-dots at various nanofiber diameters . . . .. 46. 4.6. Photon-counting for guided and radiation modes . . . . . . . . . . . .. 47. 4.7. Photon-correlations between guided and radiation modes . . . . . . .. 50. 4.8. Dependence of channeling efficiency on fiber size parameter . . . . . .. 52. 4.9. Conclusion . . . . . . . . . . . . . . . . . . . . . . . . . . . . . . . . .. 53. 5 Determination of Fluorescence Quantum Efficiency of Single Quantum Dots. 54. 5.1. Introduction . . . . . . . . . . . . . . . . . . . . . . . . . . . . . . . .. 54. 5.2. Experimental procedures . . . . . . . . . . . . . . . . . . . . . . . . .. 55. 5.3. Definition of quantum efficiency . . . . . . . . . . . . . . . . . . . . .. 57. 5.4. Fluorescence photon-count rate and normalized photon correlations .. 57. 5.5. Parameters for single q-dots on an optical nanofiber with diameter of 400 nm . . . . . . . . . . . . . . . . . . . . . . . . . . . . . . . . . . .. 60. 5.6. Distribution of quantum efficiency for single q-dots . . . . . . . . . .. 60. 5.7. Radiative and non-radiative decay rates of single q-dots . . . . . . . .. 61. 5.8. Discussion . . . . . . . . . . . . . . . . . . . . . . . . . . . . . . . . .. 62. 5.9. Conclusion . . . . . . . . . . . . . . . . . . . . . . . . . . . . . . . . .. 62. 6 Azimuthal Distribution of Quantum Dots on Nanofiber. 64. 6.1. Introduction . . . . . . . . . . . . . . . . . . . . . . . . . . . . . . . .. 64. 6.2. Experimental procedure . . . . . . . . . . . . . . . . . . . . . . . . .. 64. 6.3. Photon-counting for guided and radiation modes . . . . . . . . . . . .. 65.
(11) 6.4. Product of channeling efficiency and quantum efficiency . . . . . . . .. 67. 6.5. Parameters for q-dots on optical nanofibers . . . . . . . . . . . . . . .. 68. 6.6. Discussion . . . . . . . . . . . . . . . . . . . . . . . . . . . . . . . . .. 68. 6.7. Conclusion . . . . . . . . . . . . . . . . . . . . . . . . . . . . . . . . .. 69. 7 Conclusions and Future Prospects. 70. 7.1. Conclusions . . . . . . . . . . . . . . . . . . . . . . . . . . . . . . . .. 70. 7.2. Future Prospects . . . . . . . . . . . . . . . . . . . . . . . . . . . . .. 72. References. 75. A Degradation of Single Quantum Dots. 87. B Certificate of Analysis. 89. B.1 CoA for Lot Number: 834674 . . . . . . . . . . . . . . . . . . . . . .. 89. B.2 CoA for Lot Number: 898274 . . . . . . . . . . . . . . . . . . . . . .. 90. Acknowledgements. 91. List of Publications Related to the Thesis. 93.
(12) List of Tables. 3.1. Parameters for q-dots on optical nanofibers . . . . . . . . . . . . . . .. 5.1. Parameters for single q-dots on an optical nanofiber with diameter of. 6.1. 37. 400 nm . . . . . . . . . . . . . . . . . . . . . . . . . . . . . . . . . . .. 60. Parameters for q-dots on optical nanofibers . . . . . . . . . . . . . . .. 68. vi.
(13) List of Figures. 1.1. Single mode propagation of field through the guided mode of the optical nanofiber . . . . . . . . . . . . . . . . . . . . . . . . . . . . . . . . . .. 3. 1.2. Modified spontaneous emission around the optical nanofiber . . . . .. 5. 2.1. Optical nanofiber preparation technique . . . . . . . . . . . . . . . .. 11. 2.2. Characteristics of optical nanofibers . . . . . . . . . . . . . . . . . . .. 13. 2.3. Physical structure of a q-dot . . . . . . . . . . . . . . . . . . . . . . .. 14. 2.4. Subpicoliter needle dispenser . . . . . . . . . . . . . . . . . . . . . . .. 15. 2.5. Inverted microscope system . . . . . . . . . . . . . . . . . . . . . . .. 16. 2.6. Procedure for depositing q-dots on nanofiber surface . . . . . . . . . .. 18. 2.7. Experimental scheme for fluorescence photon measurements . . . . .. 19. 3.1. Fluorescence photon measurements procedures through the guided modes 24. 3.2. Measurement of light-transmission factor for guided modes . . . . . .. 25. 3.3. Spatial distribution of deposited q-dots . . . . . . . . . . . . . . . . .. 26. 3.4. Single/Two q-dots fluorescence photons statistics . . . . . . . . . . .. 28. 3.5. Single/Two q-dots fluorescence photons statistics . . . . . . . . . . .. 29. 3.6. Emission spectrum of a single q-dot . . . . . . . . . . . . . . . . . . .. 31. 3.7. Theoretical model for photon emission process of q-dots . . . . . . . .. 32. vii.
(14) 3.8. Intensity dependence of photon correlation recovery rates . . . . . . .. 34. 3.9. Photon-count rate histogram . . . . . . . . . . . . . . . . . . . . . . .. 35. 3.10 Intensity dependence of photon-count rates . . . . . . . . . . . . . . .. 36. 4.1. Single-mode fluorescence photon measurements procedures from a single/twodots . . . . . . . . . . . . . . . . . . . . . . . . . . . . . . . . . . . .. 4.2. 42. Measurements of fluorescence light-transmission factors for guided and radiation modes . . . . . . . . . . . . . . . . . . . . . . . . . . . . . .. 43. 4.3. Effect of nanofiber as a cylindrical lens . . . . . . . . . . . . . . . . .. 45. 4.4. Deposition of single/two q-dots at various nanofiber diameters . . . .. 46. 4.5. Photon-counting for guided and radiation modes . . . . . . . . . . . .. 47. 4.6. Photon-counting for guided and radiation modes . . . . . . . . . . . .. 49. 4.7. Schematic diagram of the experiment for measuring the photon correlations between the guided and radiation modes . . . . . . . . . . . .. 4.8. 50. Typical observed normalized photon-correlations between the guided and radiation modes . . . . . . . . . . . . . . . . . . . . . . . . . . .. 51. 4.9. Dependence of channeling efficiency on fiber size parameter . . . . . .. 52. 5.1. Single q-dots fluorescence photon measurements procedures . . . . . .. 55. 5.2. Definition of quantum efficiency . . . . . . . . . . . . . . . . . . . . .. 57. 5.3. Fluorescence photon-count rate and normalized photon correlations .. 58. 5.4. Intensity dependence of photon correlations and photon-count rates .. 59. 5.5. Distribution of quantum efficiency for single q-dots . . . . . . . . . .. 60. 5.6. Radiative and non-radiative decay rates of single q-dots . . . . . . . .. 61. 6.1. Photon-counting for guided and radiation modes . . . . . . . . . . . .. 66. 6.2. Product of channeling efficiency and quantum efficiency . . . . . . . .. 67. 7.1. Single q-dot in nanofiber cavity . . . . . . . . . . . . . . . . . . . . .. 73. A.1 Typical fluorescence photon-count rate as a function of time before degradation of the q-dot. . . . . . . . . . . . . . . . . . . . . . . . . .. 87.
(15) Chapter 1. Introduction. 1.1. Introduction. Single photons will be used as information carriers in quantum information processing and communication (QIPC) and a quantum network will have quantum nodes, where single photons will be stored, and quantum channels for transport of single photons [1– 3]. A physical implementation of a quantum network would consist of single quantum emitters representing the quantum nodes and single mode optical fibers providing the quantum channels. The high fidelity transport of single photons in the single mode optical fibers will also enable entanglement generation between distant nodes which is another key ingredient of QIPC [1, 4, 5]. So apart from controlled manipulation of single photons, channeling of single photons into a single mode optical fiber is crucial requirement for realization of QIPC. Single photon generation can be realized by manipulating single quantum emitters. For this purpose various quantum emitters like trapped atoms/ions [6–12], molecules [13–17], colour centers in diamond [18–20], mesoscopic quantum well [21], quantum dots [22–32] have been investigated. However, efficient production of single photons is useless without efficient collection and efficient transport. In order to real1.
(16) Chapter 1. Introduction. 2. ize a deterministic and efficient single photon source, strong light-matter interaction is crucial requirement. For this purpose many novel ideas have been proposed using a variety of micro/nano boundary conditions [33, 34]. Examples include micropillar cavities (∼78%) [29], plasmonic metal nano-wires (∼60%) [34], photonic crystal cavities [35], photonic crystal fiber (∼ objective lens with a NA of 0.82) [36] , and solid immersion lens (∼18%) [37]. However, in these techniques the subsequent channeling of single photons into a single-mode fiber would reduce the actual collection efficiency. For example, in micropillar cavity experiemnts channeling efficiency into a single mode fiber is of the order (14-40%) [38]. In the view of ability to directly channel the single photons into the single-mode fiber, tapered optical fibers with sub-wavelength diameter known as optical nanofibers are particularly promising. It has been theoretically predicted that, by positioning the emitter on the nanofiber surface, one can channel the photons into the nanofiber guided-modes with an efficiency higher than 20% [39,40], and moreover, fibers can be tapered adiabatically to keep the light transmission into the single-mode fiber higher than 90% [41,42]. In this thesis we demonstrate the efficienct single photons collection from single q-dots using an optical nanofiber.. 1.2. Optical nanofibers for quantum optics. Recently, optical nanofibers have attracted considerable attention in the field of quantum optics. Our group proposed a novel idea for realizing slow light propagation in a thin optical fiber by utilizing the electromagnetically induced transparency (EIT) properties of the surrounding medium [43]. The fabrication of such optical nanofibers with diameters down to 50 nm has been demonstrated by Mazur’s group [44]. Russell’s group demonstrated spectrally-broad single-mode supercontinuum generation using the unique property of propagating field confinement in micron and submicron tapered fibers [45]. Also due to the evanescent tail of the guided-modes, tapered optical fibers with micrometer diameters have shown promise application as efficient light couplers to high Q micro-resonators such as silica microspheres and microtoroids [46]..
(17) 1.2. Optical nanofibers for quantum optics. 3. Our group and Arno’s group demonstrated the realization of optical nanofibers by adiabatically tapering the single mode optical fibers [41, 42]. Some of the unique properties of such optical nanofibers are described below.. 1.2.1. Confinement of the field around the optical nanofiber. a). Evanescent Region Nanofiber Guided Field. b). Vacuum Clad nclad=1 Silica Core ncore=1.45. 2a. λ λ. Figure 1.1: (a) Single mode propagation of field through the guided mode of the optical nanofiber. These are vacuum clad fiber and a part of the field exist outside the fiber. (b) The normalized intensity profile versus the normalized radial distance (r/a) for 850 nm light propagating through the nanofiber with radius 200 nm (black curve) and 400 nm (red curve).. A nanofiber is made of a silica core and the cladding is vacuum. This large difference between the refractive indices (∼0.45) makes the nanofiber different from conventional fibers. The core of the original fiber is almost vanishing and it can support only a single fundamental mode (HE11 ) [47]. The field in the guided mode is strongly confined in space and a part of the field lies outside the fiber in the evanescent region. The fiber mode characteristics depend on the fiber radius (a) and the propagation light wavelength (λ). Figure 1.1(a) shows a schematic diagram of the nanofiber guided mode with a part of the field propagating outside the nanofiber. The field in the evanescent region.
(18) Chapter 1. Introduction. 4. decays exponentially outside the nanofiber. Figure 1.1(b) shows the intensity distribution of 850 nm light guided through fibers with radius 200 nm and 400 nm. It is clear that for smaller radius a major part of the intensity lies outside the fiber.. 1.2.2. Modified spontaneous emission of a quantum emitter around the optical nanofiber. In free-space, the quantum emitters have isotropic spontaneous emission. As a result the emission probability in a specific propagation mode is very low. However, one can manipulate the photonic environment to enhance the spontaneous emission into a specific mode. It can be understood from the Fermi’s Golden rule Γij ∝ |Dij |2 ρ(νij ),. (1.1). where Γij is the rate of spontaneous emission (i.e. the probability of emission), Dij is a matrix element that connects the excited and lower energy levels and is determined by the wavefunctions associated with those levels, ρ(νij ) is the density of the optical field at the transition frequency or the photon mode density. The control of fluorescence decay rate through photon mode density was first discussed by Purcell at microwave frequencies [48]. Here the photon mode density is modified by using the optical nanofiber. Due to strong field confinement around the optical nanofiber, the spontaneous emission rate of the emitter is modified. An appreciable amount of spontaneous emission from an emitter can be channeled into guided modes of the nanofiber [39, 40]. A detailed theoretical investigation of modified spontaneous emission of a multilevel Cs-atom around a nanofiber can be found in Ref. [40]. Here we show the results for an incoherently pumped (randomly oriented dipoles) two-level system. It is assumed that the fiber has a cylindrical silica core of radius a and the refractive indices of the fiber and the vacuum cladding are n1 =1.45 and n2 = 1, respectively..
(19) 1.2. Optical nanofibers for quantum optics. 5. γr. a). γg. ηc =. b). 25. γ g( HE. 11 ). γ r + ∑ γ g(i ). Channeling Efficiency, ηc [%]. Channeling Efficiency, ηc [%]. Emitter. i. 20 15 10 5 0. 1. 2.5 1.5 3.0 2.0 Fiber size parameter [k0a]. 3.5. c). 25 20 15 10 5 0. 0. 400 200 300 100 Emitter-fiber-surface distance [nm]. Figure 1.2: Modified spontaneous emission around the optical nanofiber. (a) Schematic diagram showing a quantum emitter on the nanofiber surface. (b) ηc as a function of the size parameter (k0 a). The optimum channeling efficiency (∼ 23%) is at a fiber size parameter of around 1.44, which corresponds to a fiber diameter (2a) of around 360 nm for the emission wavelength (λ) of 800 nm. (c) ηc as a function of emitter-fiber-surface distance.. Figure 1.2(a) shows a schematic diagram, where a quantum emitter (two-level) sits on the fiber surface. The channeling efficiency (ηc ) into the nanofiber-guided modes can be expressed as follows: (HE ). ηc =. γg 11 P , γr + γgi. (1.2). i (HE11 ). where γg. is the decay rate into the fiber fundamental mode. γr is the decay rate P i γg is the decay rate into the all possible guided modes. into the radiation modes. i. Figure 1.2(b) shows the ηc as a function of the size parameter (k0 a). The calculation has been performed, by assuming a silica refractive index of 1.45 and emitter sits on the fiber surface. One can readily see that ηc decreases as fiber diameter.
(20) Chapter 1. Introduction. 6. increases. The optimum channeling efficiency (∼ 23%) is at a fiber size parameter of around 1.44, which corresponds to a fiber diameter (2a) of around 360 nm for an emission wavelength of (λ) 800 nm. Figure 1.2(c) shows ηc as a function of the emitter-fiber-surface distance. One can readily see that ηc drops as the distance from the fiber surface in the radial direction increases. One can see that a maximum ηc can be realized at the fiber surface.. 1.2.3. Optical nanofibers as a tool for fluorescence measurements. Optical nanofibers have been attracting considerable attention in the field of quantum optics, especially in quantum information science. Many works have been reported so far using laser-cooled atoms. Channeling of fluorescence photons into the guided modes of the nanofiber has been demonstrated [41], and photon correlations from single atoms have been measured systematically through nanofiber guided modes [49]. Photon correlations for atoms have been measured and analyzed by varying atom numbers from less than one to several [50, 51]. The fluorescence emission spectrum has been measured for a few atoms through the guided mode by combining optical-heterodyne and photon-correlation methods [52, 53]. Various schemes have been proposed for trapping atoms around the nanofiber [54–56], and trapping has been experimentally demonstrated [57, 58] using a dipole-trapping method via twocolor laser-fields [55]. However, the channeling efficiency of fluorescence photons into the guided modes, has not been measured yet, although results published so far imply a reasonable correspondence to the theoretical predictions [41]. One reason that it is difficult to measure the channeling efficiency is due to the fact that atoms are not on the nanofiber surface and the atom-surface distance could not be estimated accurately. For example, in single atom detection experiments, the photon correlations show antibunching behavior for shorter time scales [49, 51]. At longer time scales, photon correlation show a decay revealing the dwell time of the single atom because the atom moves out of the observation region..
(21) 1.3. Summary of thesis. 7. However, in order to measure the channeling efficiency into the nanofiber guided modes, one crucial requirement is to extend the photon emitters from atoms to solidstate emitters, such as semiconductor q-dots. The q-dots are more convenient than atoms or ions in various aspects. For example, they do not have to be trapped like atoms, working at room temperature, desired emission wavelength by tuning its size, and easy to incorporate in photonic structures. The q-dots can serve as an efficient single-photon sources, which will be useful for applications in quantum information science.. 1.3. Summary of thesis. As discussed above optical nanofibers provide various possibilities for manipulating single photons and promise to become a useful tool in quantum information processing. The main aim of this thesis is to develop single photon source using q-dots on optical nanofibers. We demonstrate the efficient channeling of fluorescence photons from single q-dots into the guided modes of the optical nanofiber. For the experimental demonstrations, we developed a method to deposit single colloidal core-shell type q-dots systematically and reproducibly along the optical nanofiber. Chapter 2 presents the experimental techniques for optical nanofiber preparation, and deposition of q-dots, and detection are also discussed. Chapter 3 presents the experimental investigation of fluorescence photon emission characteristics for single q-dots using optical nanofibers. The absolute fluorescence photon-count rates channeled into the guided modes of nanofiber and the normalized photon correlations at various excitation laser intensities are measured. The results were able to obtain the product between the fluorescence photon channeling efficiency and the quantum efficiency of the single q-dots. Chapter 4 presents the experimental investigation of the efficient channeling of fluorescence photons from single q-dots into the guided modes of optical nanofiber by measuring the photon-count rate through the guided and radiation modes simultaneously. We obtain a maximum channeling efficiency to be 22.0 (±4.8)% at a fiber.
(22) Chapter 1. Introduction. 8. diameter of 350 nm for an emission wavelength of 780 nm. Chapter 5 presents the experimental determination of the fluorescence quantum efficiency for one q-dot very accurately by assuming the theoretical values for the channeling efficiency from the product of the channeling efficiency and the quantum efficiency. Chapter 6 presents the experimental investigation of the azimuthal position of the q-dots on the upper surface of the nanofiber and its effect on the channeling efficiency estimation. Chapter 7 presents conclusions and the possible extensions of the nanofiber work..
(23) Chapter 2. Experimental Techniques. We performed experiments by depositing colloidal core-shell type CdSeTe (ZnS) qdots on an optical nanofiber surface. Optical nanofibers are realized by adiabatically tapering single mode optical fibers using a heat and pull technique. In order to manipulate single photons, one crucial requirement is to isolate a single emitter from an ensemble. Here, we use a subpicoliter needle dispenser to deposit individual qdots from a q-dot solution onto the optical nanofiber surface. For precise alignment of the needle to the optical nanofiber, we use an inverted microscope system. Using this procedure, we can deposit single q-dots systematically and reproducibly along the optical nanofiber. The q-dots are excited through a microscope objective lens. Fluorescence photons are observed at the end of the single-mode fiber for the guided modes and through the microscope objective lens for the free space (radiation) modes. Details of the experimental procedures are described below.. 2.1. Experimental realization of optical nanofibers. We use tapered fiber technology to realize optical nanofibers. Using a heat and pull technique, commercial single-mode optical fibers are adiabatically tapered down to 9.
(24) Chapter 2. Experimental Techniques. 10. sub-wavelength diameters [41, 51, 53]. The nanofiber is located at the central part of the tapered optical fiber. Adiabatic tapering is necessary to maintain single-mode propagation and minimum transmission loss. The general single-mode condition [47] p for an optical fiber is given by the parameter V = 2πa n2core − n2clad , where a is the λ fiber radius, λ is the wavelength of the propagating light, ncore and nclad are the refractive indices of core and cladding of the fiber, respectively. The central part of the tapered fiber supports only the fundamental-mode (HE11 ). However, the tapered region can support higher order modes, which results in loss in the thinnest region. The adiabatic tapering ensures that the transition of fundamental core-mode to fundamental cladding-mode takes place without any loss of power to higher order cladding-modes [59–62]. There are various heating and pulling techniques for adiabatic sub-micron tapering. Examples include gas-flame heating (Hydrogen/Oxygen) [44], CO2 laser heating [59, 63], and microfurnaces [64]. We use a commercial optical fiber coupler manufacturing machine (NTT-AT, Model FCI-7011) for fabrication of tapered optical fibers. This device employs a microfurnace technique, which is based on a resistive ceramic heater [65,66]. Figure 2.1(a) shows a photograph of the production machine. The production machine consists of a main-unit and a control-unit. The inset shows the main-unit components in detail. The main-unit consists of a ceramic heater, pulling stages, and fiber clamps. The control-unit consists of a heater power supply, digital multimeter, and controller. All functions of the fabrication system are computer controlled. The whole system is installed inside a clean booth equipped with a high-efficiency particulate air (HEPA) filter based air cleaner (Airtech, SS-MAC). A nanofiber cell (fiber holder, shown in inset of Fig. 2.1(a)) is used to catch the nanofiber after preparation. The nanofiber cell is specially designed for our experiments to protect the nanofiber from dust and also to be compatible with the inverted microscope system..
(25) 2.1. Experimental realization of optical nanofibers. 11. (a) PC. Clean Room Control Unit. LD Ceramic Heater Clamps Nanofiber Nanofiber Cell. Pulling Stage. PD Main Unit. (b) SEM. (c). Precision End Cutter Fiber & Bobbin. Fusion-splicer. Fiber Stripper. Figure 2.1: Optical nanofiber preparation. (a) Fabrication system with main-unit and control-unit inside a clean room. The laser diode (LD) and photo diode (PD) are for making transmission measurements. The inset shows the main-unit components in detail. (b) Scanning electron microscope (SEM) for the nanofiber diameter measurements. (c) Some of the important tools used for nanofiber fabrication as well as for the experiments.. Figure 2.1(b) shows a photograph of the scanning electron microscope (SEM) (Keyence, VE-9800), which is used for the nanofiber diameter measurements. Figure 2.1(c) shows a photograph of important tools, which are used for nanofiber fabrication as well as for the experiments. A fiber stripper is used for removing the acrylate coating on the fiber with a high precision. A precision end cutter is used for cleaving the fiber perpendicularly. A fusion splicer is used for splicing between the single mode.
(26) Chapter 2. Experimental Techniques. 12. fibers with a low loss. To realize optical nanofibers, we begin with commercial single mode optical fibers (SMF) (Fujikura, SM 10/125, cutoff wavelength=1.3 µm, mode field diameter=9 µm). The SMF diameter is around 250 µm with the acrylate coating. We chemically remove the outer coating with acetone to avoid any scratches on the fiber. After chemical stripping the fiber diameter is 125 µm which includes both core (∼ 10µm) and cladding. We use a two-step pulling process for adiabatic tapering. For each step the temperature and the pulling speed are chosen to realize the required diameter and minimize optical loss. In the first step the fiber diameter is reduced from 125 µm to a few tens of µm and in the second step the diameter is reduced to sub-micron. In this sub-micron region, the core essentially vanishes and the cladding of the original fiber becomes the core of the nanofiber. During the fabrication process, in order to monitor losses, the transmission through the fiber is monitored continuously by sending light at 800 nm through the fiber and detecting it with a photo diode (PD).. 2.1.1. Characteristics of optical nanofibers. Optical nanofibers are characterized by measuring their transmission and diameter profile. The tranmission characteristics are measured for different nanofiber diameters, using a fiber-coupled superluminescence light emitting diode (SLED) at a wavelength of 800 nm. The measurement procedure is as follows: The original SMF has a cut-off wavelength of 1.3 µm. Therefore, to measure the single-mode transmission, we fusion spliced another single-mode fiber with a cut-off wavelength of 557 nm before the tapered fiber. Typical transmission values are shown in Fig. 2.2(a). One can readily see that transmission drops as the diameter becomes thinner. For fiber diameters of 300-400 nm, we could realize transmission of 85-95%. The transmission value are reproducible within measurement error..
(27) 2.1. Experimental realization of optical nanofibers. Transmission [%]. 100. a). b). 80. SEM IMAGE. 60 40 20. DIAMETER= 300 nm. 0 450. c). 400 300 350 Fiber diameter [nm]. 250. Fiber diameter [nm]. 800 600 400 200 0. d) 1000. 1000 Fiber diameter [nm]. 13. -8 -6 -4 -2 0 2 4 6 8 Length along the fiber axis [mm]. 800 600 400 200 0. -8 -6 -4 -2 0 2 4 6 8 Length along the fiber axis [mm]. Figure 2.2: Characteristics of optical nanofibers. (a) The measured transmission for different diameters of the nanofibers, using a fiber-coupled superluminescence light emitting diode (SLED) at 800 nm. (b) A typical SEM image of a nanofiber with diameter of 300 nm. (c) and (d) are diameter variation along the nanofiber axis with minimum diameter of 400 and 300 nm respectively. The different curves correspond to different nanofiber samples.. The diameter profiles of the optical nanofibers are measured using a SEM with a resolution of around 10 nm. Figure 2.2(b) shows a typical SEM image of the nanofiber region with a diameter of 300 nm. Figure 2.2(c) and (d) show the diameter profiles of the optical nanofibers with minimum diameters of 400 and 300 nm respectively. In each plot, the different curves correspond to different nanofiber samples prepared under the same production conditions. One can clearly see the minimum diameters are reproducible within the measurement error. The minimum diameter is approximately uniform for 2 mm along the fiber axis. In the tapered region, the fiber diameter varies.
(28) Chapter 2. Experimental Techniques. 14. along the fiber axis by around 100 nm/1mm. The total tapering length is around 9 cm, which ensures the adiabatic tapering condition. Ambiguity of the diameter measurements is estimated to be around 6%. For the experiments presented in this thesis nanofiber diameters of 300-400 nm were used. However, using the present technique, production of fibers with diameters down to 100 nm is possible.. 2.2 2.2.1. Precise spatial control of q-dots on optical nanofibers Semiconductor q-dots. Core (CdSeTe) Shell (ZnS) Polymer 7-10 nm Figure 2.3: Physical structure of a q-dot. The layers represent the distinct structural elements of the q-dot nanocrystal, and are roughly to scale.. For the experiments in this thesis, we use core-shell type colloidal q-dots emitting at around 790 nm (Invitogen, Q21371MP) [67]. Figure 2.3 shows the physical structure of the q-dot schematically. The q-dots are essentially made from nanocrystals of a semiconductor material (CdSeTe), which are covered with an additional semiconductor layer (ZnS) known as a shell to improve their chemical and optical properties. This core-shell material is further coated with a polymer layer that allows facile dispersion of the q-dots in aqueous solutions with retention of their optical properties. The typical size of the q-dots is around 7-10 nm..
(29) 2.2. Precise spatial control of q-dots on optical nanofibers. 2.2.2. 15. Subpicoliter needle dispenser 100. Needle diameter [µm]. a). NEEDLE HOLDER SREW. NEEDLE HOLDER. 1 2 3 4 5. 80 60 40 20 0. UPPER LEVEL. b). 0. 0.2 0.4 0.6 0.8 Needle length [mm]. 1.0. LOCK SCREW. NEEDLE KNOB NEEDLE. HEIGHT ADJUSTED KNOB. NEEDLE. RESERVOIR. GLASS-TUBE HOLDER SREW. NANOFIBER CELL. SOLUTION. RESERVOIR. 17 µm. 200 µm. Figure 2.4: Subpicoliter needle dispenser. (a) Photograph of the needle dispenser. The inset shows a schematic illustration of the needle and liquid reservoir. (b) Diameter variation along the length of the needle. The needle tip diameters are between 5-27 µm. The different curves correspond to different needles.. We use a subpicoliter needle dispenser (Applied Micro Systems, ND-2000) [68] for depositing colloidal q-dots on the nanofiber surface. Figure 2.4(a) shows a photograph of the needle dispenser. The dispenser consists of a tapered glass-tube (liquid reservoir) and a needle. The inset shows a schematic illustration of the needle and liquid reservoir. Once the needle tip passes through the tapered glass-tube, it carries a small amount of liquid at its edge. However, droplet size is determined by the tip size of the needle. Typical needle diameter profiles are shown in Fig.2.4(b). The needle tip diameters are between 5-27 µm. We use these kind of needles for depositing.
(30) Chapter 2. Experimental Techniques. 16. small amounts (on the order of subpicoliter) of q-dot solution onto the nanofiber surface. For precise alignment in the vertical (z -axis) direction, the needle tip position is computer-controlled with a resolution of 250 nm.. 2.2.3. Inverted microscope system (a) RIGHT VIEW EYEPIECE (10X). (c). DISPENSER X-Y STAGE. BS LASER. FILTER TURRET. OPTICAL PATH KNOB. OPTICAL PATH SELECTOR (EYE/R/L/AUX). (b). X-Y STAGE. OL. OBJECTIVE LENS (OL). NOSEPIECE. SAMPLE. SHUTTER LEVEL FILTER BLOCK ADDRESS. PORT. BS ILLUMINATOR. CCD. FOCUS KNOB. FM. FIBER. EYEPIECE. LEFT VIEW. (d). FILTER BLOCK PORT. SIDE PORT. PORT (CCD). CELL. STAGE RING. LAMP (INTENSITY CONTROL). Figure 2.5: Inverted microscope system. (a) Right-side view of the microscope with X -Y stage and needle dispenser in a clean room. The needle dispenser is used to deposit q-dots. (b) Left-side view of the microscope. A CCD-camera is used for observe the scattered light from the nanofiber. (c) A schematic diagram of the microscope system. OL, BS, and FM denote objective lens, beam splitter, and flipper mirror, respectively. (d) Detailed view of the nanofiber cell.. We used an inverted microscope (Nikon, Eclipse Ti-U) [69] with a high precision computer-controlled X -Y stage (Sigma koki, FC-401G) [70] for precisely aligning the.
(31) 2.2. Precise spatial control of q-dots on optical nanofibers. 17. needle to the optical nanofiber. Figures 2.5(a) and (b) show photographs of right and left side views of the microscope respectively. Figure 2.5(c) shows a schematic diagram of the microscope system. The microscope system is customized with two input ports consisting of two beam splitters (BS) (60 (T):30 (R)). One port is used for illumination and the other is used for excitation. We can switch between the two input ports by selecting the appropriate BS. The microscope system is equipped with three output ports for observation. One port is used for fluorescence observation and the other two ports are used for viewing the sample through a CCD camera and through an eyepiece. We can switch between these three ports by selecting the optical path using a flipper mirror (FM). The microscope is equipped with five objective lenses (5X, 10X, 20X, 40X, 100X) of different magnifications. The OL is used for both the excitation and fluorescence observation. Figure 2.5(d) shows the nanofiber cell fixed onto the X -Y stage for the observation. The stage can move up to ±15 mm from the center with a resolution of 50 nm.. 2.2.4. Procedure for depositing q-dots on the nanofiber surface. Figure 2.6 shows a schematic diagram of the experiment. For depositing the q-dots on the nanofiber, we pick up the central part of the tapered optical fiber by focusing on both sides at a fiber thickness of ∼ 1 µm of the tapered optical fiber. The needle dispenser is fixed to another x -y stage on top of the microscope as shown in Fig 2.5(a). The needle axis is adjusted to coincide with the axis of the microscope using the x -y stage. The inset shows a typical SEM image of the needle tip with a diameter of 17 µm, which we use for the present experiments. Regarding the deposited solution, we use a diluted q-dot solution. The original q-dot solution (5× 1015 dots/cm3 ) is diluted by pure water to reduce the concentration to 2 × 1013 dots/cm3 . Once the needle tip passes through the tapered glass-tube, it carries a small amount of q-dot solution at its edge. In order to deposit a single or a few q-dots on the nanofiber, the needle-tip position is adjusted so that the q-dot solution at its.
(32) Chapter 2. Experimental Techniques. 18. tip just touches the nanofiber. This method was found to give optimal results. To confirm that the drop is touching the nanofiber, laser light derived from a He-Ne laser is sent through the nanofiber and scattered light through the microscope objective is observed using a CCD-camera. The above procedure allows q-dots to be deposited precisely on the nanofibers. Using a Mass Flow Controller (Horiba Stec, PE-D20), we flow dust-free dry-nitrogen gas to evaporate the residual solvent on the nanofiber. Thus, the q-dots reside on the fiber surface. The dry-nitrogen flow is maintained for the whole experimental period of several days to protect the q-dots from oxygen gas. SEM IMAGE OF NEEDLE TIP. Subpicoliter needle dispenser He-Ne Laser. 17 µm. Z. OL. Inverted Microscope CCD. BS. ILLUMINATOR FM. Figure 2.6: Schematic diagram of the experiment. The inset shows a typical SEM image of the needle tip with a diameter of 17 µm, which we use for the present experiments. The nanofiber is located at the central part of a tapered optical fiber. A subpicoliter needle dispenser and an inverted microscope are used for depositing the q-dots on the nanofiber. For dropping confirmation, laser light from a He-Ne laser is sent through the nanofiber and scattered light through the microscope objective is detected by a CCD camera. OL, BS, and FM denote objective lens, beam splitter, and flipper mirror, respectively..
(33) 2.3. Procedure for fluorescence photon measurements. 2.3. 19. Procedure for fluorescence photon measurements. Figure 2.7 shows the experimental scheme for fluorescence photon measurements. After depositing q-dots on the nanofiber, we perform fluorescence measurements. We use a cw diode-laser at a wavelength of 640 nm to excite q-dots. Using the OL (40X, NA= 0.6), the excitation beam is focused on to the nanofiber.. MMF. FC1. SMF2 SMF1. SMF1 SMF2. S1. S2. FL1. MMF. FL2. APD1 MMF. OL BS CCD. FC2. SMF1. SMF1. OMA. NPBS. APD2. Pico Harp. Hanbury Brown-Twiss. LASER. FM. MMF. FL3. FC3. APD3. Figure 2.7: Schematic diagram of the experimental setup. The inset shows the optical nanofiber and microscope system. OL, BS, FM, FL and NPBS denote objective lens, beam splitter, flipper mirror, filter and non-polarizing beam splitter, respectively. SMF, FC, and MMF denote single-mode fiber, fiber coupler, and multimode fiber, respectively. LD, APD, and OMA denote laser diode, avalanche photodiode, and optical multichannel analyzer, respectively.. The fluorescence photons emitted from q-dots are channeled to the guided modes of the nanofiber and are detected through the single mode optical fiber. In order to guarantee the observation through the fundamental mode (HE11), the tapered single mode fiber (SMF1) [cut-off wavelength:1.3 µm] is fusion spliced to another singlemode fiber (SMF2) [cut-off wavelength:557 nm] at both ends as shown in Fig.2.7.
(34) Chapter 2. Experimental Techniques. 20. (S1 , S2). At both ends of the fiber, the fluorescence light is filtered out from the scattered excitation laser light by using a color glass filter (HOYA, R-72). At one end of the fiber, the photon correlations are measured using a conventional Hanbury Brown-Twiss setup [71], in which the fluorescence light beam is split into two using a 50:50 non-polarizing beam splitter (NPBS), and the split beams are re-coupled into multi-mode fibers and detected by fiber-coupled avalanche-photodiodes (Perkin Elmer, SPCM-AQR/FC). Arrival times of photons at both APDs are recorded using a two-channel single-photon-counter (Pico Quant GmbH, Pico-Harp 300). The photon correlations are later derived for delay times of up to ±1 ms from the recorded arrival times for the two channels. At the other end of the fiber, the fluorescence emission spectrum is measured using an optical-multichannel-analyzer (OMA) (Andor, DV420A-OE). Regarding the radiation modes, fluorescence photons are collected by the OL and observed at the right-side port of the microscope. We couple fluorescence photons into a multimode fiber using a Five Axis Fiber Aligner (New Focus, 9091-M), and detect them using a fiber-coupled avalanche-photodiode. A set of two filters (HOYA, R70/R72) is used to reject the scattered laser light.. 2.3.1. Avalanche photo-diodes. In order to detect fluorescence from a single/few q-dots, a high sensitivity detector is needed. We use a silicon avalanche photodiode (APD) (Perkin Elmer, SPCM-AQRFC) with a circular active area of diameter 180 µm that achieves a peak photon detection efficiency of more than 65% at 650 nm. The photodiode is both thermoelectrically cooled and temperature controlled, ensuring stabilized performance. The APD module has a fiber FC input. A 35 ns wide transistor-transistor logic (TTL) pulse is generated when a photon is detected. However, there is a dead time of 50 ns between pulses. The quantum efficiency of detection is 60 % at our fluorescence wavelength of 800 nm. The APD module has a saturation count of around 5×106 /s..
(35) 2.3. Procedure for fluorescence photon measurements. 2.3.2. 21. Counting board. The counting board functions as a photon counter when combined with a single photon counting module (SPCM). We use a commercial photon counting-board (Hamamatsu, M8784), which is capable of counting input signals with no dead time. The board is installed in a PC and controlled by a Lab View based software program.. 2.3.3. Time-correlated single photon-counting system. We use a commercial Time-Correlated Single Photon-Counting System ( Pico Quant GmbH, Pico-Harp 300), which can operate in various modes to adapt to different measurement needs. For our experimental purpose we use it in Time-Tagged-TimeResolved (TTTR) mode, in which we can record the arrival times of each photon event in two independent channels. Each channel has a dead time of around 90 ns. However, the cross channel time resolution is around 4 ps. We derive photon correlations up to a delay-time of ±1 ms from the record using analysis software, which reveals information about the fundamental second-order correlation function. We have performed photon-correlation measurements to determine the photon statistics from q-dots, and the excited state lifetime of the q-dots.. 2.3.4. Optical-multichannel analyzer. We use a CCD (Andor, DV420A-OE) based grating spectrometer for measuring the emission spectrum. We record the emission spectrum from a single/few q-dots with a resolution of 0.5 nm..
(36) Chapter 3. Fluorescence Measurements through the Guided modes of Optical Nanofiber. 3.1. Introduction. Single photon manipulation is one of the major issues in contemporary quantum optics, especially in the context of quantum information technology. For this purpose many novel ideas have been proposed. The key point of the ideas is to achieve strong confinement of the field using micro/nano boundary conditions. One major trend is to use various designs of high-Q micro-structured resonators [33]. Recently, other than the micro-resonators, new type of nano-structured systems have been proposed and demonstrated. Examples include plasmonic metal nano-wires [34] and sub-wavelength diameter silica fibers [49]. Sub-wavelength diameter silica fibers, termed as optical nanofibers, have been the subject of many investigations typically using the laser-cooled Cs-atoms [41, 49–53]. However, in order to extend the optical nanofiber method to real applications, such as single-photon source, one crucial requirement is to extend the photon emitters from atoms to solid-state emitters, such as semiconductor q-dots. In this Chapter, we. 22.
(37) 3.2. Experimental procedures. 23. experimentally investigate the fluorescence photon emission characteristics of single q-dots on an optical nanofiber using photon correlation spectroscopy. Using colloidal CdSeTe (ZnS)-nanocrystals as q-dots, we obtain fluorescence photon channeling efficiency into the nanofiber guided modes of higher than 9.4 ± 3%. We also describe a method to deposit single q-dots systematically and reproducibly on optical nanofibers.. 3.2. Experimental procedures. Figure 3.1 illustrates the schematic diagram of the experimental setup. The nanofiber is located at the central part of a tapered optical fiber produced by adiabatically tapering a commercial single mode optical fiber using a heat and pull technique [41]. The diameter of the nanofiber is measured using a SEM before and after the optical experiments. The diameter of the nanofiber is around 400 nm and is uniform for 2 mm along the fiber axis. The uncertainty in the diameter measurement is estimated to be 6%. The transmission through the tapered fiber is around 90%. A fiber-coupled SLED at a wavelength of 800 nm is used for the transmission measurements by splicing the fiber output to the optical nanofiber and measuring its output.. 3.2.1. Quantum dots deposition on the optical nanofiber. We use core-shell type colloidal CdSeTe (ZnS) q-dots having an emission wavelength around at 792 nm and the fluorescence quantum efficiency of 72% [72, 73](see Appendix B). For depositing q-dots on the nanofiber, we use the subpicoliter needle dispenser. The detailed deposition method was discussed in the Chapter 2. Using such a method q-dots are deposited periodically at 8 positions on the nanofiber by shifting the nanofiber along the x -axis in 20 µm steps. The transmission of the nanofiber drops to 81% after these procedures..
(38) Chapter 3. Fluorescence Measurements through the Guided modes of Optical Nanofiber. 3.2.2. 24. Fluorescence photon measurements procedures through the guided modes SMF FC1 MMF. OMA. FL1. SMF. Y X. FL2. OL. Inverted BS Microscope CCD. NPBS. 640 nm LD FM. FC2. MMF. APD1 APD2 MMF. Photon Correlator. Hanbury Brown-Twiss. Figure 3.1: Schematic diagram of the experiment. The q-dots are excited using a cw diode-laser at a wavelength of 640 nm. The fluorescence photons from q-dots channeled into the guided mode of the nanofiber are detected through the single mode optical fiber. At one end of the single mode fiber the photon arrival times are recorded by using a two-channel single-photon-counter, and at the other end the fluorescence emission spectrum is measured using optical multichannel analyzer (OMA). OL, BS, FM, FL, and NPBS denote objective lens, beam splitter, flipper mirror, and non-polarizing beam splitter, respectively. FC, SMF, and MMF denote fiber coupler, single-mode fiber, and multimode fiber, respectively. APD and LD denote avalanche-photodiode and laser diode respectively.. The q-dots are excited using a cw diode-laser at a wavelength of 640 nm. The excitation beam is focused on to the nanofiber through the microscope objective lens with a spot size of 5 µm FWHM. The fluorescence photons emitted from q-dots are channeled into the guided mode of the nanofiber and are detected through the single mode optical fiber. At both ends of the single mode fiber, the fluorescence light is filtered out from the scattered excitation laser light by using a color glass filter (FL1, FL2; HOYA, R-72). At one end of the single mode fiber, the photon correlations are measured using a conventional Hanbury Brown-Twiss setup [71], in which the fluorescence light beam is split into two using a 50:50 non-polarizing beam splitter (NPBS), and the split beams are re-coupled into multi-mode fibers and detected by fiber-coupled avalanche-photodiodes APD1 and APD2 (Perkin Elmer,.
(39) 3.2. Experimental procedures. 25. SPCM-AQR/FC). Arrival times of photons at both APDs are recorded using a twochannel single-photon-counter (Pico Quant GmbH, Pico-Harp 300), and the arrival timing resolution is 300 ps including the response times of the APDs. The photon correlations are derived for delay times of up to 1 ms from the recorded arrival times for the two channels. Additionally, photon-count rates for each APD are obtained from the same data. At the other end of the single mode fiber, the fluorescence emission spectrum is measured using an optical-multichannel-analyzer (Andor, OMA).. 3.2.3. Measurement of light-transmission factor for guided modes SLED 800 nm. SMF. NPBS. SMF FL2. MMF FC2. APD1. Figure 3.2: Conceptual diagram of the experiment. The κg value is the lighttransmission factor from the nanofiber region to the APD1-position.. Figure 3.2 illustrates the experimental concept. In order to obtain the absolute number of photons channeled into the guided modes, the light-transmission efficiency from the nanofiber region to the APD-detector is important. We use the photoncount rates for APD1. We denote the efficiency as κg , and the value was measured to be κg =31.0(±1.3)%. The measurement procedure is as follow: The SLED output is fusion spliced to the FL1 end, and the output power is measured at the APD1position. The input power to the optical nanofiber is measured by cleaving the SMF before entering into the tapered fiber. The measured value is consistent with a value calculated as a product of transmission factors of optical nanofiber (81%), color-glass filter (FL2; 83%), NPBS (51%), and the coupling efficiency into the multi-mode fiber (FC2; 90%)..
(40) Chapter 3. Fluorescence Measurements through the Guided modes of Optical Nanofiber. 3.3 3.3.1. 26. Emission behavior of q-dots on optical nanofibers Spatial distribution of deposited q-dots. 20. Photon-count rate [kcps]. 6. 15. 5 4. 10. 3 1. 5. 2 7 8. 0 -20. 60 20 40 0 80 100 120 140 Position along the optical nanofiber [µm]. Figure 3.3: The observed fluorescence photon-count rates as a function of the focusing point along the nanofiber. The excitation laser intensity was kept at 15 W/cm2 . One can clearly see eight sharp peaks signal along the nanofiber with a spacing of 20 ± 5 µm, which corresponds well to the q-dot placement on the nanofiber. The signals are numbered from 1 to 8.. Figure 3.3 shows the observed fluorescence photon-count rates as the focusing point along the nanofiber was scanned with a speed of 2 µm/s. The excitation laser intensity was kept at 15 W/cm2 . Eight sharp peaks in the signal are observed along the nanofiber, with a spacing of 20 ± 5 µm, which corresponds to the expected q-dot placement distribution on the nanofiber. The peaks are numbered from 1 to 8. It is readily seen that each observed signal shows much sharper width than the focused beam size of 5 µm and some of the signals show double or triple peak structure. This.
(41) 3.3. Emission behavior of q-dots on optical nanofibers. 27. behavior may be understood as being due to the blinking of q-dots [74, 75] when the laser beam passes over the q-dot position.. 3.3.2. Single/Two q-dots fluorescence photons statistics. Fluorescence photon measurements are carried out for each signal position by varying the excitation laser intensity from 20 to 900 W/cm2 . The measurement time for each position is 5 min. The photon-count rate and photon correlation are displayed in Figs. 3.4 and 3.5 for all positions. The excitation intensity is 50 W/cm2 for the positions of 1 to 6, and 8, and 130 W/cm2 for the position 7. The left columns show photon-count rates as a function of time. We average the photon-count over the time bin size of 17 ms and plot the histogram of fluorescence photon-count as a function (2). of time. The right columns show the normalized photon correlations gN (τ ) for delay time τ up to ±600 ns with a time resolution of 1 ns. The normalized correlations were calculated by deriving the self-coincidences in each channel independently up to 1 ms. Regarding the photon-count rates in the left columns, the on/off-behaviors are clearly observed for all positions. We have analyzed the on/off-times with a resolution down to nanosecond time scale. The on/off-times follow a power law behavior as discussed in Ref. [76, 77] and we interpret the on/off-behavior as the blinking of the q-dots. For positions 1, 2, 3, 5, 7, and 8 the blinking shows a single-step behavior, and for positions 4 and 6 a two-step behavior is observed. The single-step blinking means that the number of q-dot is just one at that position, while the two-step blinking corresponds to the two q-dots. Regarding position 8 as shown in Fig. 3.5, the offperiod was quite long compared to the on-period, and we could not obtain reliable data at this position. In the following, we describe the results for positions from 1 to 7. The normalized correlations in the right column clearly show anti-bunching behaviors for all positions. The correlations at the anti-bunching dip are close to zero; they are 0.042, 0.031, 0.035, 0.034, and 0.050 for positions 1, 2, 3, 5, and 7, respectively, but for positions 4 and 6 the values are 0.19 and 0.45 respectively. Since the nor-.
(42) Chapter 3. Fluorescence Measurements through the Guided modes of Optical Nanofiber. 40. Position 3. 28. 1.5. 30. 1. 20 0.5. 10. Position 5. Position 7. 0 1.5. Normalized correlation. Photon-count rates [kcps]. Position 4. 0 70 60 50 40 30 20 10 0 50 40 30 20 10. 1 0.5 0 1.5 1 0.5. 0. 0. 30. 1.5. 20. 1. 10. 0.5. 0. 0. 20. 40. 60. Time [s]. 80. 100. 0 -600 -400 -200 0. 200 400 600. Delay time [ns]. Figure 3.4: The left column shows the photon-count rates as a function of time and (2) the right column shows the normalized photon correlation gN (τ ) for four positions (3, 4, 5, and 7). Excitation intensity is 50 W/cm2 for the positions of 3, 4, and 5, and 130 W/cm2 for the position 7. The red curves show the exponential fitting of the normalized photon correlations..
(43) 3.3. Emission behavior of q-dots on optical nanofibers. 1.5. 30. Position 2. 1. 20. 0.5. 10. Normalized correlations. Photon-count rates [kcps]. Position 1. 29. 0 40 30 20 10 0 60. 0 1.5 1 0.5 0 1.5. 50 40. Position 6. 1. 30 20. 0.5. 10. Position 8. 0. 20. 40. 60. Time [s]. Photon-count rate [kcps]. 0. 80. 100. 0 -600 -400 -200 0. 200 400 600. Delay time [ns]. 25 20 15 10 5 0. 0. 200. 600 400 Time [s]. 800. Figure 3.5: The left column shows the photon-count rates as a function of time and (2) the right column shows the normalized photon correlations gN (τ ) for three positions (1, 2, and 6). Excitation intensity is 50 W/cm2 for the positions of 1, 2, 6, and 8. At the position 8 the off-period was quite long compared to the on-period, so we could not obtain reliable photon correlation data. The red curves show the exponential fitting of the normalized photon correlations..
(44) Chapter 3. Fluorescence Measurements through the Guided modes of Optical Nanofiber. 30. (2). malized correlation for N -emitters [78] at τ = 0 is expressed as gN (0) = (N − 1)/N, the number of q-dots for each position can be obtained to be one for positions 1, 2, 3, 5, and 7. Regarding position 4, we obtain the number to be two, although the dip value is much smaller than the theoretical value of 0.5. This may imply that one dot may be less emissive than the other. Regarding position 6, we obtain the number to be two, since the value is close to the theoretical value of 0.5. These results are consistent with blinking behavior. The dip values are summarized in Table 3.1. The anti-bunching signals are fitted by single exponential curves (red curves). The obtained recovery times are 60, 48, 55, 83, 25, 108, and 43 ns for the positions 1, 2, 3, 4, 5, 6, and 7, respectively.. 3.3.3. Emission spectrum of a single q-dot. The emission spectrum was measured for each q-dot deposition. In Fig. 3.6, a typical spectrum for one (black, red) and two (blue) dots obtained at positions 7, 3, and 6 are displayed, respectively. The spectrums were obtained with an integration time of 5 min with an excitation intensity of 50 W/cm2. The center wavelengths (λc ) are 746, 796, and 826 nm, and the spectral widths (∆λ) are 52, 52, and 72 nm FWHM for the positions 7, 3, and 6, respectively. However, in the case of two q-dots spectral width depends on the center wavelength of each q-dot. The measured λc and ∆λ values for all the positions are listed in Table 3.1. The center wavelengths are distributed over a range of 80 nm from 746 to 826 nm. The measured spectrum width is narrower than the ensemble average width value of 82 nm [72] (see Appendix B). The mechanism of the broadening may be understood due to spectral diffusion and exciton-phonon interactions which have been discussed for similar q-dots [79, 80]..
(45) 3.4. Single/Two q-dots photon statistics data analysis. Normalized intensity [a.u.]. 1. 31. Position 7 [Single] Position 3 [Single] Position 6 [Two]. 0.8 0.6 0.4 0.2 0 500. 600. 800 900 700 Wavelength [nm]. 1000. 1100. Figure 3.6: The emission spectrum for a single (black, red) and two (blue) q-dots measured at positions 7, 3, and 6, respectively. The excitation intensity is 50 W/cm2. The single q-dot spectrum (red) reveals, center wavelength is at 796 nm and the spectral width is 52 nm FWHM.. 3.4. Single/Two q-dots photon statistics data analysis. 3.4.1. Theoretical model for photon emission process of qdots. Figure 3.7 illustrates the schematic energy-level diagram for the fluorescence photon emission process of q-dots. The scheme is essentially an incoherent two-level system, since photo-excited electrons quickly relax to the emission state |1i [23, 75]. The excited-state population N1 can be described by the following rate equation, N1 N˙ 1 = αIN0 − , τ. (3.1).
(46) Chapter 3. Fluorescence Measurements through the Guided modes of Optical Nanofiber. 32. where N0 is the ground-state population, αI is the excitation rate at laser intensity I, and 1/τ is the total decay rate of the excited state |1i. Assuming a single q-dot (N0 + N1 = 1) and initial condition N1(t = 0) = 0, the temporal evolution of the excited-state population is readily derived as follows:. 1. αI. 1/τ 0. Figure 3.7: Schematic energy-level diagram for the photo-emission process of q-dots. The gray non-radiative relaxation process is assumed to be very fast.. t αI N1(t) = 1 − exp(− ) , αI + 1/τ T 1/T = αI + 1/τ,. (3.2). where 1/T is the intensity-dependent decay-rate. Then, the photon emission rate into the nanofiber guided modes can be expressed as follows: αI 1 ηq ηc nf iber (t) = N1 (t) × × ηc = τr τ αI + 1/τ. t 1 − exp(− ) , T. (3.3). where 1/τr denotes the radiative decay rate of the excited state |1i and ηc the channeling efficiency of the fluorescence photons into the guided modes. It should be mentioned that the radiative decay rate is related to the quantum efficiency (ηq ) of q-dot by ηq = (1/τr )/(1/τ ). The observable photon-count rate by the APD (nobs ) can be obtained from the photon-emission rate (nS ) under the stationary condition by multiplying the trans-.
(47) 3.4. Single/Two q-dots photon statistics data analysis. 33. mission efficiency κg determined in Section 3.2.3 and the quantum efficiency (ηAP D ) of the APD. nS and nobs can be readily obtained from Eq. (3.3) as follows. A factor of 1/2 is introduced due to the fact that the channeled photons are detected only for one direction of the nanofiber. Note that the rates are dependent on the excitation laser intensity.. nobs (I) = ηAP D κg. αI αI nS (I) ηq ηc = ηAP D κg = nobs (∞) 2 2τ αI + 1/τ αI + 1/τ. (3.4). where nobs (∞) = ηAP D κg ηq ηc /2τ .. 3.4.2. Intensity dependence of photon correlation recovery rates. Since the anti-bunching recovery process is essentially a population evolution of the excited state, the recovery rates can be expressed by the intensity-dependent decayrate 1/T in Eq. (3.2). In Fig. 3.8, observed recovery rates for positions 3, 4, 5, and 7 are plotted versus the excitation laser intensity. One can readily see the linear dependence. The data points are fitted by the least-mean squares method, and the fitted results are shown by solid lines. As seen in Eq. (3.2), the intercept at the zero-intensity gives the decay rate of the excited state 1/τ . Obtained decay times are listed in Table 3.1. The decay times are distributed over the range of 150 ± 50 ns for the seven positions..
(48) Chapter 3. Fluorescence Measurements through the Guided modes of Optical Nanofiber. Recovery rate, 1/T [ns-1]. 0.25. 34. 3 4 5 7. 0.20 0.15 0.10 0.05 0. 0. 400 200 600 800 Excitation laser intensity, I [W/cm2]. Figure 3.8: The observed anti-bunching recovery rates (1/T ) for different excitation laser intensities at positions 3 (red circles), 4 (blue triangles), 5 (black squares) and 7 (green triangles). The solid lines show linear fits to the data.. 3.4.3. Photon-count rate histogram. Figure 3.9 (a) and (b) show the fluorescence photon-count rates as a function of time at positions 3 and 4, respectively. The right column of Fig. 3.9 shows the photoncount rate histogram for the fluorescence trace for the whole measurement time with a counting interval of 1 kcps. The histogram (right column of Fig. 3.9(a)) reveals a clear two peak structure, which corresponds to “on/off” state occurrence probabilities. By fitting the “on” state occurrence probability histogram with a Gaussian profile [81], we obtain nobs (I ) to be 27.1 (±3.6) kcps. In a similar fashion photon-count rates are obtained at each intensity and at each deposition..
(49) 3.4. Single/Two q-dots photon statistics data analysis. 35. Photon-count rate [kcps]. Position 3. a). 40 30 20 10 0. 20. 0. 60 40 Time [s]. 80. 100. 500 1000 2000 0 Number of occurences. 100. 0. Photon-count rate [kcps]. Position 4. b). 70 60 50 40 30 20 10 0. 0. 20. 60 40 Time [s]. 80. 100 200 300 400 Number of occurences. Figure 3.9: The left column shows the photon-count rates as a function of time and the right column shows the photon-count rate histogram. (a) and (b) corresponds to positions 3 and 4, respectively. The red solid curve is a fit of a Gaussian profile.. 3.4.4. Intensity dependence of photon-count rates. Figure 3.10 shows the fluorescence photon-count rate versus excitation intensity measured at the four positions 3, 4, 5, and 7. One can readily see saturation behavior for all plots. We fitted the measured results with Eq. (3.4). We fixed the parameters α and 1/τ to the values determined from the intensity dependence of the anti-bunching recovery rates. The adjustable parameter is nobs (∞). The fitted results are shown by dashed curves and the obtained parameter nobs (∞) is listed in Table 3.1 for the seven positions. We obtain ηq ηc from the obtained parameters using the relation.
(50) Chapter 3. Fluorescence Measurements through the Guided modes of Optical Nanofiber. 36. nobs (∞) = ηAP D κg ηq ηc /2τ , where ηAP D is assumed to be 60%. The obtained values. Photon-count rate, nobs(I) [kcps]. are shown in Table 3.1.. 40 30 3 4 5 7. 20 10 0. 0. 600 800 400 200 Excitation laser intensity, I [W/cm2]. Figure 3.10: The observed fluorescence photon-count rates for different excitation intensities at the four positions 3 (red circles), 4 (blue triangles), 5 (black squares) and 7 (green triangles). The observed photon-count rates are fitted (dashed curves) using Eq. (3.4)..
(51) 3.5. Parameters for q-dots on optical nanofibers. 3.5. Parameters for q-dots on optical nanofibers. Table 3.1: Position 1 (2) gN (0) 0.042 ±0.002 λc [nm] 798 ∆λ [nm] 58 τ [ns] 100 ±40 nobs (∞) 30.0 [kcps] ±1.0 ηq ηc 0.033 ±0.014. 3.6. 37. Parameters for q-dots on optical nanofibers. 2 3 4 5 6 0.031 0.035 0.190 0.034 0.450 ±0.002 ±0.002 ±0.001 ±0.001 ±0.014 820 796 784 798 826 68 52 52 60 72 195 130 126 140 190 ±90 ±20 ±13 ±45 ±50 20.0 38.4 42.0 39.8 44.7 ±0.8 ±1.2 ±2.5 ±0.9 ±5.6 0.043 0.054 0.057 0.060 0.094 ±0.020 ±0.010 ±0.009 ±0.021 ±0.030. 7 0.050 ±0.002 746 52 130 ±50 45.7 ±2.2 0.064 ±0.028. Discussion. Table 3.1 summarizes the measured results for the seven positions. The second row (2). shows the gN (0) values. One can readily see that a single q-dot is deposited for (2). positions 1, 2, 3, 5, and 7 where gN (0) 1. On the other hand, two q-dots are deposited for positions 4 and 6. For position 4, the situation is described in Section (2). 3.3.2. Regarding position 6, gN (0) value is close to 0.5. This means that the deposited two q-dots behave almost the similar to each other. This situation provides a contrast to that for position 4. The observations reveal that single q-dot can be deposited reproducibly using the present method. On average of depositing a single q-dot with this method was about 60%. As seen for the third row to sixth row of Table 3.1, the obtained parameter values show some variations for the seven positions. These variations are reasonable, since in the present experiments, we picked up one or two q-dots at each position from a huge number of q-dots in the q-dot solution (5× 1015 dots/cm3 ), and the physical properties of individual q-dot are expected to show variation around a mean value. We can also obtain the channeling efficiency of emitted photons into the nanofiberguided modes from the derived ηq ηc values shown in the seventh row of Table 3.1. The ηq ηc values show a variation from 0.033 to 0.094. We suspect that this variation reflects.
(52) Chapter 3. Fluorescence Measurements through the Guided modes of Optical Nanofiber. 38. the variation of the quantum efficiency of q-dots, since the ηc value can be assumed to be the same for the seven positions which are distributed along the nanofiber within a range of 150 µm. The manufacturer of the q-dots gives a value for the quantum efficiency ηq of 72% [72, 73]. It should be noted that the value is a mean value measured for a liquid sample which contains a huge number of q-dots (5× 1015 dots/cm3 ). The ηq value for a single q-dot is expected to distribute around the mean value. The obtained highest ηq ηc value corresponds to a q-dot which has the highest quantum efficiency among the measured q-dots. Assuming the highest ηq value to be 100%, we obtain the ηc value. Note that the value gives the lower limit for the channeling efficiency, since the ηq value of 100% is highest possible value. Thus, from the obtained highest ηq ηc value of 0.094 at position 6, we obtained the lower limit of ηc value to be 9.4 ± 3%.. 3.7. Conclusion. We have experimentally investigated the fluorescence photon emission characteristics for single q-dots by using an optical nanofiber. We have demonstrated that single qdots can be deposited systematically and reproducibly along an optical nanofiber. For single q-dots on an optical nanofiber, we measured the fluorescence photon-count rates channeled into the nanofiber guided modes and the normalized photon correlations, by varying the excitation laser intensity. We have obtained a value for the fluorescence photon channeling efficiency into the nanofiber guided modes of higher than 9.4 ± 3%..
(53) Chapter 4. Efficient Channeling of Fluorescence Photons into the Guided modes of Optical Nanofiber. 4.1. Introduction. Efficient collection of fluorescence photons from a single emitter into a single-mode fiber is a major challenge in the context of quantum information science. For that purpose various novel techniques have been proposed so far. Examples include micropillar cavities [29], photonic crystal cavities [35], solid immersion lens [37], and plasmonic metal nanowires [34]. However, in these techniques the subsequent coupling of fluorescence photons into a single-mode fiber may reduce the actual collection efficiency. For example, in micropillar cavity experiments channeling efficiency to a single mode fiber is of the order (14-40%) [38]. In the view of the ability to directly channel the fluorescence photons into the single-mode fiber, optical nanofibers are particularly promising. It has been theoretically predicted that, by positioning the emitter on the nanofiber surface, one can channel the fluorescence photons into the nanofiber-guided modes with an efficiency higher than 20% [39, 40], and moreover, fibers can be tapered adiabatically to keep. 39.
(54) Chapter 4. Efficient Channeling of Fluorescence Photons into the Guided modes of Optical Nanofiber. 40. the light transmission into the single-mode fiber higher than 90% [41, 42]. As discussed in the Chapter 3, the measurements through the guided modes presented so far were able to determine a product between the channeling efficiency (ηc ) and the fluorescence quantum efficiency of single q-dot (ηq ) [82, 83]. These two parameters have different physical nature in the sense that, the value of ηq intrinsically depends on the q-dot environment via the total decay rate of its excited state, while ηc is determined by the interaction between the q-dot dipole moment and the nanofiber. However, the ηc cannot be determined without accurate information on ηq . Therfore, in this Chapter a method is devised to determine ηc by measuring the photon-count rates through the guided and radiation modes simultaneously. For measuring various diameters of the nanofiber, a maximum channeling efficiency we obtained was 22.0 (±4.8)% at a fiber diameter of 350 nm for an emission wavelength of 780 nm. The measured results completely reproduce the theoretical predictions [39,40] within experimental error.. 4.2. Experimental procedures. Figure 4.1 shows a schematic diagram of the experimental setup. Optical nanofibers are produced by adiabatically tapering commercial single-mode optical fibers (SMF1, cutoff wavelength: 1.3 µm) using a heat and pull technique [41]. The diameter of the nanofiber is measured using a SEM before and after the optical experiments. The thinnest diameter of the nanofiber is 300-400 nm, and the nanofiber diameter varies along the fiber axis by 100 nm/1 mm (data is shown in Fig.2.2). The ambiguity of the diameter measurements is estimated to be 6%. The transmission through the optical nanofiber is measured to be 90% using the SLED at a wavelength of 800 nm..
図
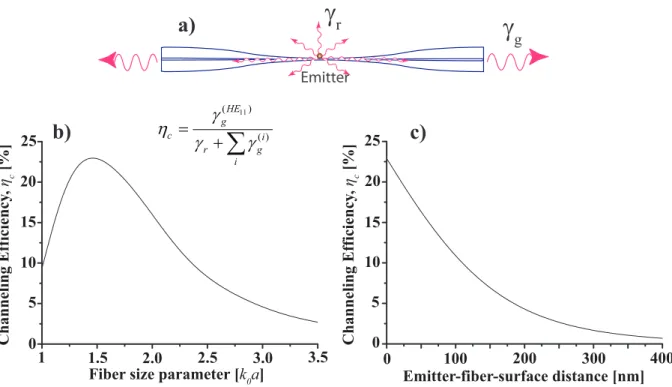
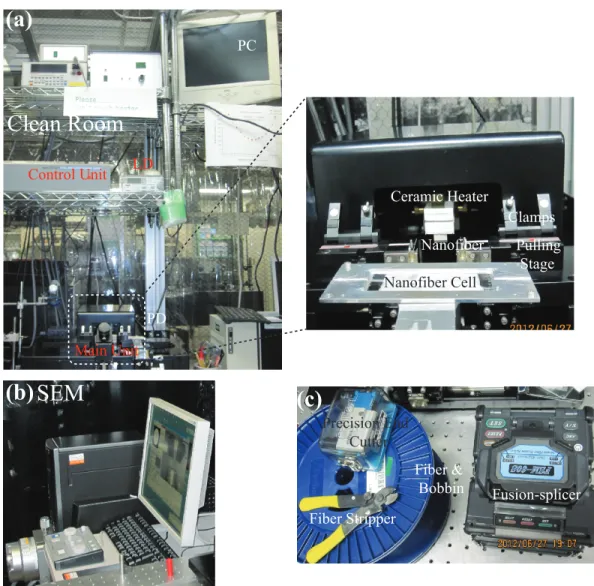
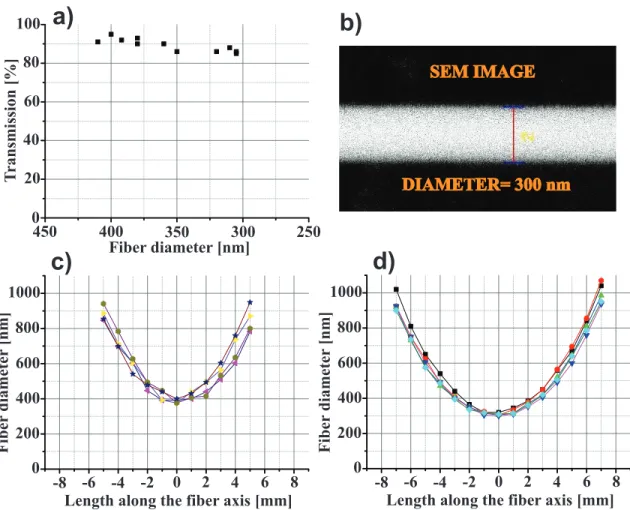
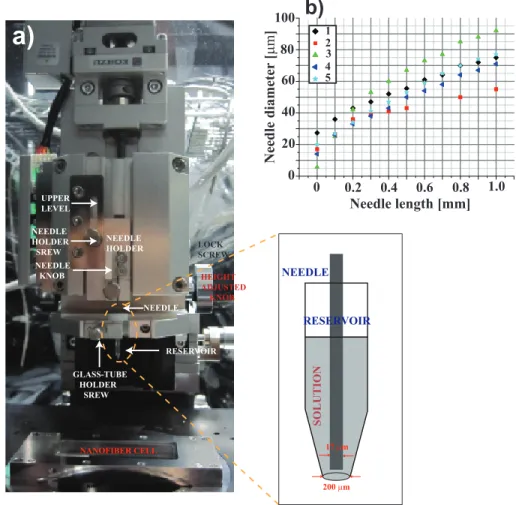
Outline
関連したドキュメント
Keywords: generalized Fokker – Planck; deterministic method; radiotherapy; particle transport; Boltzmann equation; Monte Carlo.. AMS Subject Classification: 35Q20; 35Q84; 65C05;
An easy-to-use procedure is presented for improving the ε-constraint method for computing the efficient frontier of the portfolio selection problem endowed with additional cardinality
(54) Further, in order to apply the Poisson summation formula and the saddle point method later, we consider to restrict ∆ ′′ 0 to ∆ ′ 0 of the following lemma; we will use
Equivalent conditions are obtained for weak convergence of iterates of positive contrac- tions in the L 1 -spaces for general von Neumann algebra and general JBW algebras, as well
this result is re-derived in novel fashion, starting from a method proposed by F´ edou and Garcia, in [17], for some algebraic succession rules, and extending it to the present case
It was shown in [34] that existence of an invariant length scale in the theory is consistent with a noncommutative (NC) phase space (κ-Minkowski spacetime) such that the usual
・保守点検に関する国際規格IEC61948-2 “Nuclear medicine instrumentation- Routine tests- Part2: Scintillation cameras and single photon emission computed tomography imaging”
We study the classical invariant theory of the B´ ezoutiant R(A, B) of a pair of binary forms A, B.. We also describe a ‘generic reduc- tion formula’ which recovers B from R(A, B)