Single-Inertialization Based on High-Backdrivability Control Using Equivalent Disturbance Compensator for Human Interaction Robot
全文
(2) Single-Inertialization Based on High-Backdrivability Control Using Equivalent Disturbance Compensator(Shunsuke Suzuki et al.). conventional method 2. The MNC is used for friction compensation and backdrivability are improved. The RRC is used for vibration suppression. However, both approaches regulate the inertia of the plant system. The EDC is able to realize the both single-inertialization and vibration suppression. Therefore, the human is able to feel the only load-side inertia when manipulating the robot arm in the case of direct teaching. At results, the EDC provides the higher backdrivability than conventional methods 1 and 2. This study verifies the effectiveness of the proposed approach through numerical simulation and experiments. 2.. According to the final value theorem, the value of Eq. (2) is constantly expressed as lim s s→0. where τdis (s) is defined as a step-type disturbance. Therefore, in this plant model, a constant acceleration cannot be achieved for a constant torque input. The friction on the motor side is amplified by the square of the reduction ratio and hinders the back drive. In a two-inertia system with a high reduction ratio, the reduction of friction is the first problem. 2.2 Conventional Method 1 Fig. 2 shows the block diagram of the MNC. The MNC compensates for the viscous friction on the motor side and usually employs a torsion torque sensor. However, this study does not use a torsion torque sensor. The torsion torque instead is estimated using an acceleration sensor and a force sensor based on Eq. (4).. Conventional Backdrive Control. 2.1 Two-inertia System Figure 1 illustrates a block diagram of the two-inertia system, and Table 1 lists the parameters. This study defines “load-side disturbance” as not only the environmental reaction torque, but also the friction. Equation (1) expresses the transfer function from the disturbance τdis to the torsion torque τ s , as shown in Fig. 1.. τ s (s) = τdis (s) s3 +. DM 2 JM s. Ks JL. s+. +. J M K s R2g +JL K s JL J M R2g. DM Ks JL J M. s+. DM Ks JL J M. τˆ s = τdis + aL JLn · · · · · · · · · · · · · · · · · · · · · · · · · · · · · · · · (4) Equation (5) shows the transfer functions of Giqc (s) and G sens (s). G sens (s) is the sensor dynamics, and Giqc (s) is the dynamics of the current controller, where both are approximated by a first-order low-pass filter.. · · · · · · · (1). Equation (1) also reveals that the torsion torque vibrates when a disturbance is added. The load-side velocity increases first because the load-side inertia accelerates when the disturbance torque is applied. However, the motor-side inertia does not move initially. Therefore, a fluctuation occurs in the torsion torque. Equation (2) expresses the transfer function from the disturbance τdis to the load-side acceleration aL , as shown in Fig. 1.. G sens (s) =. JL J M R2g. giqc g sens , Giqc (s) = · · · · · · · · · (5) s + g sens s + giqc. In Fig. 2, gmnc is the bandwidth of the MNC. Here, assuming that gmnc → ∞, Giqc (s) = G sens (s) = 1.0 and that there are no parameter variations, and the motor-side friction is able to be ignored, the transfer function from the disturbance τdis to the torsion torque τ s (Fig. 2) is able to be expressed by Eq. (6).. Ks DM 2 1 3 JL s + JL J M s + JL J M R2g s aL (s) =− · · · · · (2) J K R2 +J K τdis (s) s3 + D M s2 + M s g L s s + D M K s JM. aL (s) 1 = 0,· · · · · · · · · · · · · · · · · · · · · · · · · · · · · · · (3) τdis (s) s. τ s (s) = τdis (s) s2 +. JL J M. Ks JL Ks JL. +. Ks J M R2g. · · · · · · · · · · · · · · · · · · · · · · · · (6). Equation (6) indicates, that when a disturbance is applied, the torsional torque occurs in the undamped vibration mode of the secondary system. The transfer function from the disturbance τdis to the loadside acceleration aL is expressed by Eq. (7).. Fig. 1. Block diagram of two-inertia system Table 1. Definition of plant parameters variables JL τdis Iq aL ωL ωS τS DM. Load-side inertia Disturbance torque q-axis current Load-side acceleration Lade-side velocity Torsional velocity Torsional torque Motor-side viscosity. JM Rg Giqc (s) aM ωM θS Kt. Motor-side inertia Gear ratio Transfer function of current controller Motor-side acceleration Motor-side velocity Torsional angle Motor torque constant. Fig. 2. Block diagram of MNC with two-inertia system (Conventional Method 1) (10) 283. IEEJ Journal IA, Vol.10, No.3, 2021.
(3) Single-Inertialization Based on High-Backdrivability Control Using Equivalent Disturbance Compensator(Shunsuke Suzuki et al.) Ks 1 2 JL s + JL J M R2g aL (s) 1 =− =− K K s s τdis (s) JL s2 + + 2 JL. =−. J M Rg. 1 1 + J L J L s2 +. ⎛ ⎜⎜⎜ ⎜⎜⎜1 − ⎜⎝ s2 +. Ks JL Ks JL. +. Ks J M R2g. Ks JL Ks JL. +. Ks J M R2g. ⎞ ⎟⎟⎟ ⎟⎟⎟ ⎟⎠. · · · · · · · · · · · · · · (7). In Eq. (7), the second term is the vibration term. Thus, the MNC (conventional method 1) cannot realize the singleinertialization because the MNC has the vibration mode. Here, the steady state value is calculated as follows: lim s s→0. 1 aL (s) 1 =− .· · · · · · · · · · · · · · · · · · · · (8) 2 τdis (s) s J M Rg + JL. From Eq. (8), the theoretical inertia of conventional method 1 is given by aL (s) 1 −1 Conv.1 = J M R2g + JL = Jall .· · · · (9) Jeq = lim s s→0 τdis (s) s As indicated in Eq. (9), the theoretical inertia is the same as all inertia Jall of the two-inertia system. From Eq. (7), when a step disturbance is inputted to a two-inertia system with an MNC attached, the load-side inertia accelerates while oscillating. As indicated in Eqs. (7)–(9), the inertia experienced by the driver of the back drive is the total inertia converted to the load side. However, robot manipulation, such as direct teaching is affected by vibration mode and the conventional method 1 (MNC) is not suitable for single-inertialization. Therefore, when the MNC is installed, the operator of the back drive is able to operate without experiencing friction on the motor side. However, large industrial robots have large load-side and motor-side inertias, which imposes a burden on the operator. 2.3 Conventional Method 2 Figure 3 shows a block diagram of the two-inertia system with a resonance ratio controller(RRC) (11) and MNC. The RRC provides feedback on the torsional torque. Here, β represents the resonance ratio gain. When β is increased, the resonance frequency shifts to a higher frequency. The torsion-torque used by the RRC is estimated by an instantaneous state observer (ISOB) (12) . It estimates the state value instantly. In Fig. 3, the transfer function from the disturbance τdis to the torsion torque τ s is expressed as Eq. (10) when Giqc (s) = G sens (s) = 1.0, gmnc → ∞ and when no parameter variations nor estimated variable errors exist. τ s (s) = τdis (s) s2 +. +. =−. Ks β J M R2g. lim s s→0. Ks JL Ks JL. +. Ks β J M R2g. ,· · · · · · · · · · · · · (11). β 1 aL (s) 1 =− =− .· · · · · · (12) J M R2g τdis (s) s J M R2g + JL β +J β. L. Similarly, the theoretical inertia of conventional method 2 is expressed by 2 aL (s) 1 −1 J M Rg Conv.2 = Jeq + JL .· · · · · · · · (13) = lim s s→0 τdis (s) s β From Eq. (11), clearly, when a step disturbance is inputted into a two-inertia system with MNC, the load-side inertia implies accelerating while oscillating. The inertia felt by the driver of the back drive varies according to the value of β, as shown in Eqs. (12) and (13). The conventional method 2 (MNC+RRC) is also not suitable for single-inertialization. Therefore, the operator experiences a large inertia when the conventional method is applied.. · · · · · · · · · · · · · · · · · · · · · · · (10). Equivalent Disturbance Compensator (EDC) for Single-Inertialization. 3.1 Concept of EDC In previous research, the EDC is proposed to reduce the fluctuation of the torsion torque (13) (14) . The EDC realizes the torsion-torque vibration suppression compensator of the two-inertia system. Figure 4 illustrates the concept of the EDC. The EDC a torque for the motor side that is equivalent to the disturbance torque. This torque provides acceleration for the motor side equivalent to the load-side acceleration by disturbance. The torsion phenomenon does not occur owing to this disturbance. The current by the EDC is fed back to the motor-side current, that current is obtained multiplying the estimated disturbance and the gain G EDC . G EDC is indicated in Eq. (14).. Equation (10) indicates that when a disturbance is applied, the torsional torque occurs in an undamped vibration mode of the secondary system. In addition, if β is increased, this oscillation shifts to higher frequencies, and the steady-state value decreases. The transfer function from the disturbance τdis to the loadside acceleration aL is expressed by Eq. (11). ⎛ ⎞ Ks β 1 2 Ks ⎟⎟⎟ JL s + JL J M R2g aL (s) 1 ⎜⎜⎜⎜⎜ JL ⎟⎟⎟⎟ =− =− 1 − ⎜ τdis (s) JL ⎜⎝ s2 + KJLs + JKsRβ2 s2 + KJLs + JKsRβ2 ⎠ M g. 1 1 + J L J L s2 +. where the second term of Eq. (11) is the vibration term. In Eq. (11), the steady state vale is calculated as follows:. 3.. Ks JL Ks JL. Fig. 3. Block diagram of MNC+RRC (Conventional Method 2) (11). M g. 284. IEEJ Journal IA, Vol.10, No.3, 2021.
(4) Single-Inertialization Based on High-Backdrivability Control Using Equivalent Disturbance Compensator(Shunsuke Suzuki et al.). 3.2 Robustness of EDC Equation (17) shows the transfer function from disturbance τdis to the torsion torque τ s in the two-inertia system with the EDC when the EDC gain is G EDC , where ΔG EDC denotes the fluctuation from the nominal values.. τ s (s) = τdis (s) (a) Without EDC. ΔG EDC =. (b) With EDC. Fig. 4. Concept and physical meaning of Proposed Method. Ks JL (1. − ΔG EDC G sens (s)Giqc (s)) s2 +. Ks JL. +. Ks J M R2g. · · · · · · · · (17). Kt Jmn Rgn Jl · · · · · · · · · · · · · · · · · · · · · · · (18) Ktn Jm Rg Jln. In Eq. (17), the influence from the load-side disturbance torque to the torsion torque should be suppressed so that it is less than the original amplitude in the transfer function of the original two-inertia system of Eq. (6). Therefore, the relationship between Eqs. (6) and (17) is given by . Ks 1 − ΔG Ks EDC JL JL < 2 Ks K K K s + JL + JM Rs 2g s2 + JLs + JM Rs 2g < 1, · · · · · · · · · · · · · · · · · · · · · · · · · (19) ⇒1 − ΔG EDC. where Giqc (s) = G sens (s) = 1.0. Thus, the range of ΔG EDC that satisfies Eq. (19) is determined as 0 < ΔG EDC < 2. Equation (14) shows that ΔG EDC is always positive because all values related to gain design are scalar. Therefore, the EDC is able to reduce the vibration of the torsion torque when G EDC is set to the maximum expected value. 3.3 Single-Inertialization Equation (20) expresses the transfer function from the disturbance to the load-side acceleration in the case of D M = 0 from Fig. 1.. Fig. 5. Block diagram of two-inertia system with proposed method. For simplify, this section assumes that D M = 0. A block diagram of the two-inertia system with EDC is presented in Fig. 5. G EDC =. Ks 1 2 JL s + JL J M R2g aL (s) =− J K R2 +J K τdis (s) s2 + M s g L s. J Mn Rgn · · · · · · · · · · · · · · · · · · · · · · · · · · · · · · · (14) JLn Ktn. ⎛ ⎜⎜⎜ = ⎜⎜⎜⎜⎝ s2 +. The EDC provides the motor torque that is converted from the disturbance of the load side. The EDC suppresses the occurrence of the difference velocity and variation of the torsion torque. Equation (15) presents the transfer function from the disturbance torque to the torsion torque when all the parameters match the nominal value shown in Fig. 5. τ s (s) = τdis (s) =. Ks JL (1. Ks JL. +. Ks JL. s2 +. Ks JL. +. Ks J M R2g. Ks JL. +. Ks J M R2g. ⎞ ⎟⎟⎟ 1 − 1⎟⎟⎟⎟⎠ · · · · · · · · · · · · · · (20) JL. Equation (20) demonstrates that the vibration occurs on the load side during the backdrive. Moreover, it shows that the operator experiences all the inertia. However, Eq. (21) expresses the transfer function from the disturbance to the loadside acceleration in the case of Fig. 5: ⎛K ⎞ ⎜⎜⎜ s (1 − G sens (s)Giqc (s)) ⎟⎟⎟ 1 aL (s) J ⎟⎟⎟⎟ = ⎜⎜⎜⎜⎝ L − 1 ⎠ JL τdis (s) s2 + K s + K s 2. − G sens (s)Giqc (s)). s2 +. JL J M R2g. Ks JL. Ks J M R2g. s2 + (g sens + giqc )s .· · · · (15) (s + g sens )(s + giqc ). JL. J M Rg. 1 = − ,· · · · · · · · · · · · · · · · · · · · · · · · · · · · · · · · · (21) JL. Equation (15) shows the transfer function from load-side disturbance τdis to torsion torque τ s when the EDC is installed. As the bandwidths of the sensor and current control system are higher than the resonant frequency of the control system, Eq. (15) is approximated as Eq. (16).. where G sens (s) → 1 and Giqc (s) → 1. The theoretical inertia in the proposed method is calculated as the load-side inertia until the bandwidths of G sens (s) and Giqc (s). In this paper, the bandwidths of G sens (s) and Giqc (s) are 6280 rad/s (1 kHz). Thus, Eq. (21) is satisfied until the high-frequency range of 1 kHz. From Eq. (21), the theoretical inertia in the proposed method is given by aL (s) 1 −1 Prop. = JL .· · · · · · · · · · · · · · · · · (22) Jeq = lim s s→0 τdis (s) s . τ s (s) → 0.· · · · · · · · · · · · · · · · · · · · · · · · · · · · · · · · · · · (16) τdis (s) The EDC suppresses the fluctuation of the torsion torque by the disturbance under the condition of G sens (s) → 1, Giqc (s) → 1. 285. IEEJ Journal IA, Vol.10, No.3, 2021.
(5) Single-Inertialization Based on High-Backdrivability Control Using Equivalent Disturbance Compensator(Shunsuke Suzuki et al.). Fig. 7. Block diagram of Proposed Method 2 (14) Fig. 6. Block diagram of Proposed Method 1 (13). Therefore, Eqs. (21) and (22) show that the EDC realizes single-inertialization only for load-side inertia. The EDC realizes the backdrive smoothly because of the reduction in inertia. This effect appears only on the load side. The plant system is a two-inertia system when controlled from the motor side. 3.4 Control Systems Friction is not considered when describing the EDC in the previous subsection. This study proposes an EDC that performs two types of friction compensation. 3.4.1 Proposed Method 1 This method uses a friction observer. The MNC considers a two-inertia system but uses a torsion-torque sensor or sensors. The friction observer is the disturbance observer of a single inertia system. This observer does not consider the two-inertia system but is completed using only a force sensor. Therefore, this method achieves a significant cost reduction. Figure 6 shows the EDC based on the force sensor information. The friction observer subtracts the torque detected by the force sensor from the disturbance estimated by the disturbance observer. Here, gdis is the bandwidth of the disturbance observer. 3.4.2 Proposed Method 2 The control system shown in Fig. 7 is an EDC using an MNC. This control system uses both a force sensor and an acceleration sensor. 3.4.3 Analysis of Theoretical Inertias Fig. 8 shows the simulation results of load-side acceleration VS disturbance torque response of each backdrive simulation for direct teaching. As shown in the red and blue lines of Fig. 8, the conventional method 1 and 2 show a geometric similarity of linearity while having vibration. This linearity means the inertia of the controlled plant from the view of the load side and this inclination corresponds to the theoretical inertia (see Eqs. (9), (13), and (22)). Moreover, in conventional method 1 and 2, the theoretical inertia of conventional method 2 is smaller than that of the conventional method 1. This is because the conventional method 2 regulates the motor-side inertia using the RRC gain β; thus, inertia is able to be smaller. On the other hand, the proposed method 1 and 2 have a geometric similarity of linearity without vibration (see black and. Fig. 8. Simulation results of load-side acceleration VS disturbance torque (input torque) response of each backdrive simulation for direct teaching. Blue, red, green, and black lines are conventional method 1, conventional method 2, proposed method 1, and proposed method 2, respectively. green lines of Fig. 8 and Eq. (22)). Therefore, the proposed method achieves single-inertialization. In addition, the theoretical inertia of proposed method is smaller than that of two conventional methods. Therefore, the proposed method is able to realize high backdrivability. 4.. Evaluation of Direct Teaching. An experiment is performed on a human backdrive. This experiment is performed using an industrial robot and it implemented with three types of control systems, including the proposed method. The implemented control system is described as follows. • Conventional Method 1 (MNC) • Conventional Method 2 (MNC + RRC) • Proposed Method 1 (Friction Observer + EDC) • Proposed Method 2 (MNC + EDC) 4.1 Experimental Setup Figure 9 shows the industrial robot which has six axes at the time of the experiment. The control system is mounted on only the first axis, whereas the other axes are locked. Table 2 presents the parameters 286. IEEJ Journal IA, Vol.10, No.3, 2021.
(6) Single-Inertialization Based on High-Backdrivability Control Using Equivalent Disturbance Compensator(Shunsuke Suzuki et al.) Table 3. Correlation coefficient, equivalent inertia, and theoretical inertia Controller Conv. 1 Conv. 2 Prop. 1 Prop. 2. Correlation coef. 0.88 0.73 0.94 0.90. Equiv. inertia[kgm2 ] 30.2 25.1 9.68 8.55. Theoretical inertia[kgm2 ] 27.7 21.0 7.45 7.45. Table 4. Theoretical and measured values of inertia reduction rate Controller Conventional Method 2 Proposed Method 1 Proposed Method 2. Table 2. Parameters and bandwidth of an experimental machine Load-side inertia Motor-side inertia Gear ratio Torsion stiffness. 7.45 kgm2 1.27 × 10−3 kgm2 126.15 4.1 × 104 Nm/rad. Giqc (s) G sens (s) gmnc gmnc gIS OB β. Bandwidth of current controller Bandwidth of sensor Bandwidth of MNC Bandwidth of friction observer Bandwidth of ISOB Resonance ratio gain. 6280 rad/s 6280 rad/s 100 rad/s 100 rad/s 150 rad/s 1.5. Measured value[%] 17 68 72. b = Intercept L = Cumulative sum of squared errors n = Number of samples.. Fig. 9. Experimental equipment. JL JM Rg Ks. Theoretical value[%] 25 73 73. Table 3 presents the correlation coefficient of the input torque and acceleration responses, the identified equivalent inertia, and theoretical inertia. The closer this correlation coefficient is to 1, the higher is the linearity and the smaller the vibration. Table 4 presents the theoretical and measured values of the inertia reduction rate. These values are compared with the theoretical values. The theoretical values of conventional method 2 are calculated using Eq. (24). Meanwhile, the theoretical values of the proposed method are calculated using Eq. (25). Under the ideal conditions of friction compensation, no difference exists between the proposed methods 1 and 2. The inertia reduction rate of the measured value is calculated based on the equivalent inertia of conventional method 1. −1 lim s→0 s aL (s) 1 τdis (s) s Inertia reduction rate of RRC = 1 − Jall. and bandwidth of the experimental machine. Acceleration and force sensors are attached to the tip of the robot. The acceleration sensed by the acceleration sensor is converted into a joint space using the inverse Jacobian matrix whereas the force sensed by the force sensor is converted into a joint space using the transposed Jacobian matrix. The motors is controlled through servo packs using a Linux computer, and the computer control cycle is 1000 μs. 4.2 Experimental Results Figures 10 and 11 show the results of the backdrive experiment. Figure 10 illustrates the load-side acceleration versus the disturbance torque (input torque). Figure 10 displays the time response of the load-side acceleration and disturbance torque (input torque) in each method. The red lines in Fig. 10 represents inclination obtained by the least-squares method from the point cloud. The green and blue lines represent the inclinations of the total inertia and load-side inertia, respectively. The equivalent inertia shown in Fig. 10 is defined as the inertia identified by the least-squares method from the point cloud. Equation (23) defines the error. The least-squares method is used to find a and b that minimize this value.. =1−. JL +. J M R2g β J M R2g. JL + · · · · · · · · · · · · · · · · · · · · · · (24) JL Inertia reduction rate of EDC = 1 − JL + J M R2g · · · · · · · · · · · · · · · · · · · · · · (25) Figures 10(a) and 11(a) show the responses of conventional method 1. In Fig. 10(a), the inclination of the response approximates the total inertia. The MNC compensates only for friction. Figure 11(a) depicts the time response of the torque input and acceleration. In Fig. 11(a), the left axis is the acceleration response measured by the load-side acceleration sensor, whereas the right axis is the input torque measured by the force sensor, with this input torque being the external force when the robot arm is manipulated with human hands. Compared with the acceleration and input torque responses, the phase difference between both responses is nearly zero, as shown in Fig. 10(a). Therefore, as shown in Figs. 10(a) and 11(a), conventional method 1 exhibit a relationship of geometric similarity with a linear function in the magnitude between acceleration and input torque responses. Table 3 show that the correlation coefficient of conventional method 1 is 0.88. According to Eq. (6), vibration cannot be suppressed by the conventional method and thus continues.. L = Σni=1 e2i ⎞2 ⎛ ⎛ ⎞2 ⎜⎜⎜ ⎟⎟ yi + 1a xi − b ⎟⎟⎟ ⎜⎜⎜ yi + 1a xi − b ⎟⎟⎠ + ⎜⎜⎝yi − a = ⎜⎜⎝ xi − + b⎟⎟⎟⎠ 1 1 a+ a a+ a · · · · · · · · · · · · · · · · · · · · · · (23) The definitions of variables in Eq. (23) are given as follows: xi = Acceleration responses yi = Input torques a = Equivalent inertia 287. IEEJ Journal IA, Vol.10, No.3, 2021.
(7) Single-Inertialization Based on High-Backdrivability Control Using Equivalent Disturbance Compensator(Shunsuke Suzuki et al.). (a) Conventional method 1. (b) Conventional method 2. (c) Proposed method 1. (d) Proposed method 2. Fig. 10. Experimental results of load-side acceleration VS disturbance torque(input torque) response. (a) MNC (Conventional method 1). (b) MNC+RRC (Conventional method 2). (c) Friction Observer+EDC (Proposed method 1). (d) MNC+EDC (Proposed method 2). proposed method 1 exhibits a high geometric similarity between acceleration and input torque responses, as shown in Figs. 10(c) and 11(c). Table 4 presents that the theoretical values of the inertia reduction rate are 73%, whereas the inertia reduction rate from the experimental results is 69%. Figures 10(d) and 11(d) show the responses of proposed method 2. Figure 10(d) and Table 3 show that proposed method 2 has significantly reduced inertia as compared to the conventional method. Table 3 also shows the ship maneuvering coefficient is 0.90, which is higher than that of the conventional method. Equation (17) shows that the EDC exhibits a higher geometric similarity with linearity to prevent the occurrence of vibration. Table 4 indicates that the theoretical values of the inertia reduction rate are 73%, whereas the inertia reduction rate derived from the experimental results is 72%, which is close to the theoretical value.. Figures 10(b) and 11(b) illustrate the responses of conventional method 2. The equivalent inertia is reduced when Figs. 10(a) and 10(b) are compared. As shown in Table 4, the theoretical values of the inertia reduction rate are 25% and the experiment showed results of 20%. Table 3 shows that the correlation coefficient of conventional method 2 is 0.73, which is the lowest value obtained from the experiment. This value implies that the vibration increases, and the larger the value of β, the larger the vibration. However, conventional method 2 also exhibits a relationship of geometric similarity with a linear function in the magnitude between acceleration and input torque responses, as shown in Figs. 10(b) and 11(b). Figures 10(c) and 11(c) show the response of proposed method 1. Figure 10(c) and Table 3 demonstrate that proposed method 1 significantly reduces the inertia as compared to the conventional method. Table 3 also shows that the correlation coefficient is 0.94, which is the highest value. Thus, 288. IEEJ Journal IA, Vol.10, No.3, 2021.
(8) Single-Inertialization Based on High-Backdrivability Control Using Equivalent Disturbance Compensator(Shunsuke Suzuki et al.). (a) Conventional method 1. (b) Conventional method 2. (c) Proposed method 1. (d) Proposed method 2. Fig. 11. Experimental results of time response of load-side acceleration and disturbance torque(input torque). (a) MNC (Conventional method 1). (b) MNC+RRC (Conventional method 2). (c) Friction Observer+EDC (Proposed method 1). (d) MNC+EDC (Proposed method 2) Table 5. Parameters and bandwidth of an experimental machine. 5.. JL JM Rg Ks Ke De. Load-side inertia Motor-side inertia Gear ratio Torsion stiffness Environment stiffness Environment viscous friction. 4.45 kgm2 4.49 × 10−4 kgm2 121 1.86 × 104 Nm/rad 100000 Nm/rad 500 Nms/rad. Giqc (s) G sens (s) gmnc gIS OB β. Bandwidth of current controller Bandwidth of sensor Bandwidth of MNC Bandwidth of ISOB Resonance ratio gain. 6280 rad/s 6280 rad/s 100 rad/s 150 rad/s 1.5. results of environment reaction torque response in the contact with environment. In Figs. 12 and 13, black, blue, green, and red lines are conventional method 1, conventional method 2, proposed method 1, and proposed method 2, respectively. As shown in Figs. 12 and 13, simulation and experimental results are similar waveform. However, there are some difference between Figs. 12 and 13. The conventional methods 1 and 2 (MNC and MNC+RRC) have two peaks in reaction torque response. This is because that the conventional methods 1 and 2 include two-inertia parameters, especially, the motor-side inertia Jm , and torsion stiffness K s . On the other hand, the proposed methods 1 and 2 (Friction observer+EDC and MNC+EDC) have single peak because the proposed methods 1 and 2 realize the single-inertialization that consists of the load-side inertia Jl in load-side. From Figs. 12 and 13, the second peak of the conventional method 2 is lower than that of the conventional method 1. This is because the conventional method 2 uses RRC, and the RRC regulates the motor-side inertia by feeding back the RRC gain β. Second, compared with Figs. 12 and 13, the environment reaction torque at 0 s is different. This is reason why the environment reaction torque shown in simulation result is the actual environment reaction torque, but environment reaction torque shown in experimental result is the environment reac-. Evaluation of Contact Response. 5.1 Setup of Experiment and Numerical Simulation. The contact experiment is performed as a transient response of the backdrive. In this experiment, the third axis is controlled for each controller equivalent, as in the steadyresponse experiment. The other axis is locked. In the experiment, the arm of the robot contacted an iron stand at 20 rad/s (motor-side velocity). Table 5 presents the parameters and bandwidth of the experimental machine and simulation. 5.2 Experimental and Numerical Simulation Results Figures 12 and 13 show the simulation and experimental 289. IEEJ Journal IA, Vol.10, No.3, 2021.
(9) Single-Inertialization Based on High-Backdrivability Control Using Equivalent Disturbance Compensator(Shunsuke Suzuki et al.). The all methods that include the conventional and proposed methods have still remained issues, such as parameter variations. As future work, we are going to consider the compensation approach for parameter variation.. References (1). (2). Fig. 12. Simulation results of environment reaction torque response in contact with environment. (3). (4). (5). (6). (7). (8). Fig. 13. Experimental results of environment reaction torque response in contact with environment. (9). tion torque detected by a force sensor. Thus, two results have the difference waveform between Figs. 12 and 13 at 0 s. In addition, the environment reaction torque in simulation and experimental results around 0.02 s are also different. This is the effect of environmental viscosity. As described above contexts, there are a little different environment reaction torque in the simulation and experimental results, but the effectiveness of the proposed method is validated. 6.. ( 10 ). ( 11 ). ( 12 ). Conclusion. ( 13 ). This study presents an equivalent disturbance compensator (EDC) for high backdrive control. The proposed method achieves a single inertia of the two-mass system viewed from the load side by preventing the generation of torsional torque resulting from disturbance. The efficiency of the proposed method is evaluated by steady and transient responses. The steady-state response is verified that the equivalent inertia perceived by humans is reduced by manual backdrive control. It is also shown that the equivalent inertia perceived by the subject is significantly reduced and became equivalent to the load-side inertia. In the transient response, the contact with the iron environment is evaluated; the equivalent inertia is evaluated through experiments and numerical simulations. The experiments and numerical simulations showed that the proposed method operates two-inertia system as single-inertia system, even in the transient state. This enables us to verify the usefulness of the proposed method.. ( 14 ). H. Matsuki, K. Nagano, and Y. Fujimoto: “Bilateral Drive Gear—a Highly Backdrivable Reduction Gearbox for Robotic Actuators”, in IEEE/ASME Transactions on Mechatronics, Vol.24, No.6, pp.2661–2673 (2019) Y. Fujimoto, T. Kominami, and H. Hamada: “Development and Analysis of a High Thrust Force Direct-Drive Linear Actuator”, in IEEE Transactions on Industrial Electronics, Vol.56, No.5, pp.1383–1392 (2009) C.S. Cyusa and Y. Fujimoto: “Enactment-Based Direct-Drive Test of a Novel Radial-Gap Helical RotLin Machine”, in IEEE Transactions on Industry Applications, Vol.54, No.2, pp.1273–1282 (2018) H. Kaminaga, H. Tanaka, T. Amari, Y. Niwa, and Y. Nakamura: “Development of Hydrostatic Knee Power Assist Device and Its Control with Sensitivity Maximization”, in Journal of the Robotics Society of Japan, Vol.29, No.7, pp.609–618 (2011) T. Takayama, G. Chiba, and T. Omata: “Large Grasp Force Hand with High Compliance during High Speed Phase”, in Transactions of The Japan Society of Mechanical Engineers Series C, Vol.79, No.802, pp.1893–1903 (2013) T. Saito and H. Ikeda: “A Robot Actuator and a Safety Interlock System Utilizing Magneto-rheological Fluid”, in Research Reports of the National Institute of Industrial Safety, NIIS-RR-No.2003, pp.13–27 (2004) S. Yu, T. Huang, D. Wang, B. Lynn, D. Sayd, V. Silivanov, Y.S. Park, Y. Tian, and H. Su: “Design and Control of a High-Torque and Highly Backdrivable Hybrid Soft Exoskeleton for Knee Injury Prevention During Squatting”, in IEEE Robotics and Automation Letters, Vol.4, No.4, pp.4579–4586 (2019) A. Hasegawa, H. Fujimoto, and T. Takahashi: “Filtered Disturbance Observer for High Backdrivable Robot Joint”, in Proceedings in The 44th Annual Conference of the IEEE Industrial Electronics Society, IECON2018, pp.5086– 5091 (2018) S. Yamada, K. Inukai, H. Fujimoto, K. Omata, Y. Takeda, and S. Makinouchi: “Joint torque control for two-inertia system with encoders on drive and load sides”, in Proceedings in 2015 IEEE International Conference on Industrial Informatics, INDIN2015, pp.396–401 (2015) Y. Kawai, Y. Yokokura, K. Ohishi, and T. Miyazaki: “Smooth Human Interaction Control using Torsion Torque Controller and Motor-side Normalization Compensator Focusing on Back-forward Drivability”, in IEEJ Journal of Industry Applications, Vol.8, No.2, pp.322–333 (2019) K. Yuki, T. Murakami, and O. Kohei: “Vibration Control of a 2 Mass Resonant System by the Resonance Ratio Control”, in IEEJ Journal of Industry Applications, Vol.113, No.10, pp.1162–1169 (1993) T. Yoshioka, T.T. Phuong, A. Yabuki, K. Ohishi, T. Miyazaki, and Y. Yokokura: “High-performance Load Torque Compensation of Industrial Robot using Kalman-filter-based Instantaneous State Observer”, in IEEJ Journal of Industry Applications, Vol.4, No.5, pp.589–590 (2015) S. Suzuki, K. Ohishi, Y. Yokokura, and Y. Kawai: “Suppression method of Torsion Torque Response by Disturbance Torque Using Equivalent Disturbance Compensator in Tow-Inertia System”, in Joint conference of Hokuriku chapters of Electrical Society 2017, p.1 (2017) S. Suzuki, Y. Kawai, K. Ohishi, Y. Yokokura, and T. Miyazaki: “Equivalent Disturbance Compensator for robust torsion torque control of two-inertia system”, in The papers of Technical Meeting on “Mechatronics Control”, IEE Japan 2018, pp.19–24 (2018). Shunsuke Suzuki (Member) received B.E. and M.E. degrees in electrical engineering from Nagaoka University of Technology, Nagaoka, Japan, in 2018 and 2020. He is currently with YASKAWA Electric Corp., Kitakyushu, Japan. He is a member of the Institute of Electrical Engineering of Japan (IEEJ).. 290. IEEJ Journal IA, Vol.10, No.3, 2021.
(10) Single-Inertialization Based on High-Backdrivability Control Using Equivalent Disturbance Compensator(Shunsuke Suzuki et al.) Yusuke Kawai (Member) received B.E. degree in electrical engineering and the Ph.D. degree in Science of Technology Innovation from Nagaoka University of Technology, Nagaoka, Japan, in 2015 and 2020. He is currently a Postdoctoral Research Associate in Science of Technology Innovation. His research interests include motion control and Haptics. He is a member of the Institute of Electrical Engineering of Japan (IEEJ) and the IEEE Industrial Electronics Society (IEEE IES).. Toshimasa Miyazaki (Member) received the B.E. and M.E., and Ph.D. degrees all in electrical engineering, from Nagaoka University of Technology, Niigata, Japan, in 1994, 1996, and 1999, respectively. From 1999 to 2009, he was an Associate Professor with Nagaoka National College of Technology, Niigata, Japan. Since 2010, he was an Associate Professor with Nagaoka University of Technology, Niigata, Japan. Since 2020, he has been a Professor with Nagaoka University of Technology, Niigata, Japan. His research interests include motion control and powerelectronics. He is a member of the IEEE Industrial Electronics Society (IEEE IES), the Institute of Electrical Engineers of Japan (IEEJ), and the Society of Instrument and Control Engineers (SICE).. Yuki Yokokura (Member) received the B.E. and M.E. degrees in electrical engineering from Nagaoka University of Technology, Niigata, Japan, in 2007 and 2009, respectively, and the Ph.D. degree in integrated design engineering from Keio University, Yokohama, Japan. He was a Visiting Fellow at Keio University, and a Postdoctoral Fellow at Nagaoka University of Technology in 2011. Dr. Yokokura was a JSPS (Japan Society for the Promotion of Science) Research Fellow (DC2 and PD) from 2010 to 2011. He was an Assistant Professor with Nagaoka University of Technology from 2012 to 2020, and he has been an Associate Professor in 2020. His research interests include motion control, motor drive, powerelectronics, and real-world haptics.. Taiga Shinozaki (Student Member) received B.E. degrees in electrical engineering from Nagaoka University of Technology, Nagaoka, Japan, in 2020. Now he is a candidate of the M.E. degree in Electrical, Electronics and Information Engineering. His research interests include motion control and real-world haptics. He is a member of the Institute of Electrical Engineering of Japan (IEEJ).. Kiyoshi Ohishi (Fellow) received the B.E., M.E., and Ph.D. degrees in electrical engineering from Keio University, Yokohama, Japan, in 1981, 1983, and 1986, respectively. From 1986 to 1993, Prof. Ohishi was an Associate Professor with Osaka Institute of Technology, Osaka, Japan. Since 1993, he has been with Nagaoka University of Technology, Niigata, Japan. He became a Professor in 2003. His research interests include motion control, mechatronics, robotics and power electronics. He received twice “IEEJ Distinguished Paper Award” from IEEJ in 2002 and 2009, respectively. He is an IEEE Fellow member from 2015. He is a Senior AdCom Member of IEEE IES Society from 2016, and he was an AdCom Member (elected) of IEEE IES Society for 12 years from 2004. He received the Outstanding Paper Awards at IECON’ 85 and Best Paper Awards at IECON’ 02, IECON’ 04 from the IEEE Industrial Electronics Society. He is a General chair of IEEE IECON2015, AMC2010, AMC2016 and AMC2018.. 291. IEEJ Journal IA, Vol.10, No.3, 2021.
(11)
図
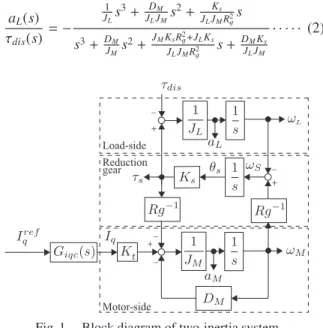
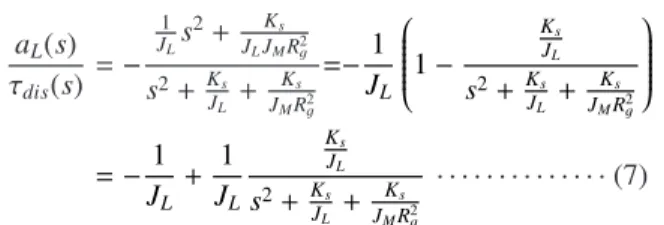
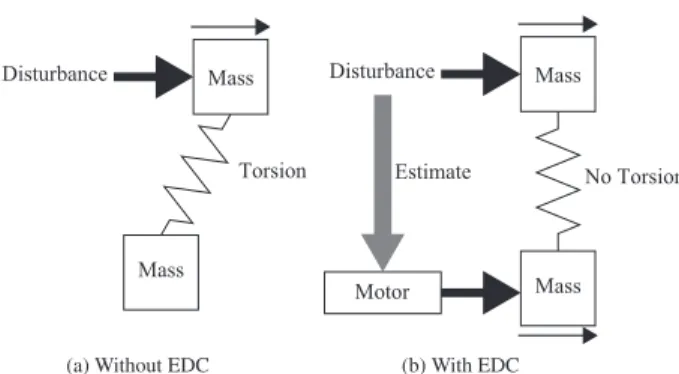
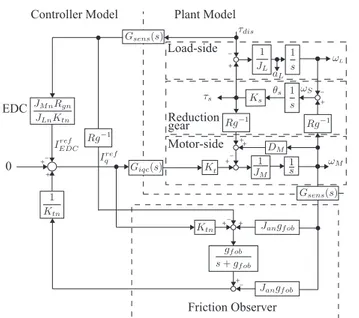
関連したドキュメント
A Study on Vibration Control of Physiological Tremor using Dynamic Absorber.. Toshihiko KOMATSUZAKI *3 , Yoshio IWATA and
and Shitani, Y., “Vibration Control of a Structure by Using a Tunable Absorber and an Optimal Vibration Absorber under Auto-Tuning Control”, Journal of Sound and Vibration, Vol.. S.,
Assume that Γ > 3γ/2 and the control bound m is large enough such that the bang arc u m starting from the north pole intersects the singular arc z 0 γ/2δ, Then for the problem
In order to improve the coordination of signal setting with traffic assignment, this paper created a traffic control algorithm considering traffic assignment; meanwhile, the link
In 2003, Agiza and Elsadany 7 studied the duopoly game model based on heterogeneous expectations, that is, one player applied naive expectation rule and the other used
A variance inequality for spin-flip systems is obtained using comparatively weaker knowledge of relaxation to equilibrium based on coupling estimates for single site disturbances..
Based on the stability theory of fractional-order differential equations, Routh-Hurwitz stability condition, and by using linear control, simpler controllers are designed to
These authors make the following objection to the classical Cahn-Hilliard theory: it does not seem to arise from an exact macroscopic description of microscopic models of