Numerical Experiment on the Fortnight Variation of theResidual Current in the Ariake Sea
全文
図
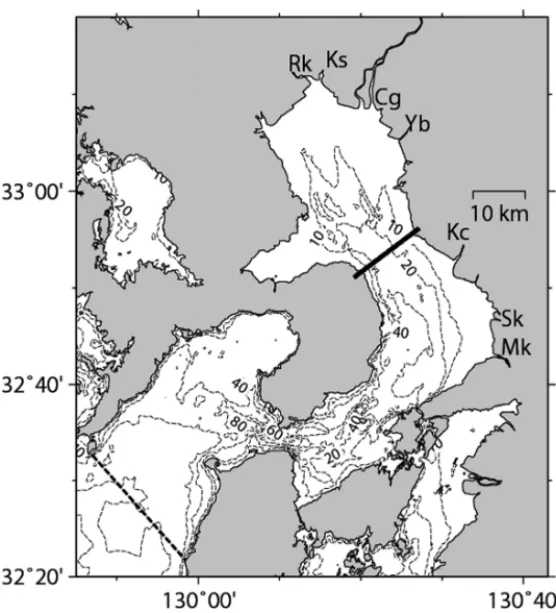
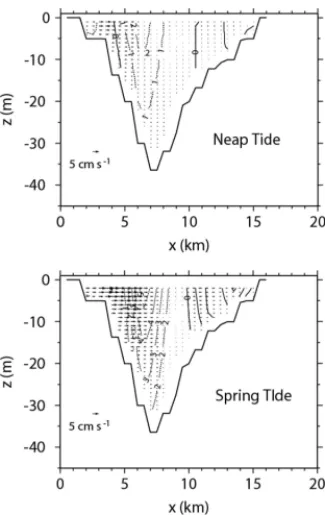
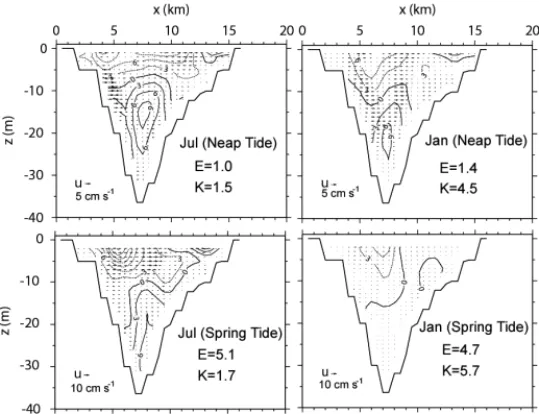
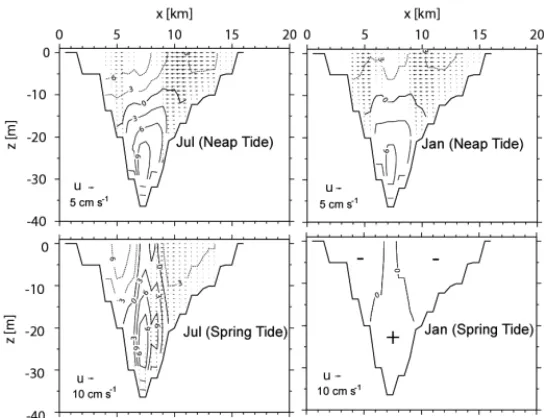
関連したドキュメント
Related to this, we examine the modular theory for positive projections from a von Neumann algebra onto a Jordan image of another von Neumann alge- bra, and use such projections
Then it follows immediately from a suitable version of “Hensel’s Lemma” [cf., e.g., the argument of [4], Lemma 2.1] that S may be obtained, as the notation suggests, as the m A
Our method of proof can also be used to recover the rational homotopy of L K(2) S 0 as well as the chromatic splitting conjecture at primes p > 3 [16]; we only need to use the
In this paper we focus on the relation existing between a (singular) projective hypersurface and the 0-th local cohomology of its jacobian ring.. Most of the results we will present
We provide an efficient formula for the colored Jones function of the simplest hyperbolic non-2-bridge knot, and using this formula, we provide numerical evidence for the
Rostamian, “Approximate solutions of K 2,2 , KdV and modified KdV equations by variational iteration method, homotopy perturbation method and homotopy analysis method,”
Section 4 contains the main results of this paper summarized in Theorem 4.1 that establishes the existence, uniqueness, and continuous dependence on initial and boundary data of a
We study the classical invariant theory of the B´ ezoutiant R(A, B) of a pair of binary forms A, B.. We also describe a ‘generic reduc- tion formula’ which recovers B from R(A, B)